Megharani Mahajan 1*
1, Department of Medicine, Hematology and Oncology, The University of Texas Health Science Center San Antonio, San Antonio, Textas, United States
E-mail:
mahajanm@uthscsa.edu
Received: 23/08/2023
Acceptance: 24/09/2023
Available Online: 26/09/2023
Published: 01/10/2023
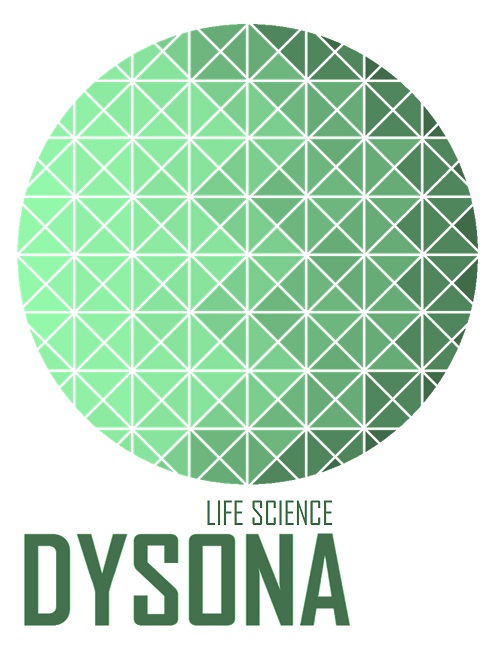
Manuscript link
http://dx.doi.org/10.30493/DLS.2023.413087
Abstract
Despite the significant progress made in the areas of early detection, treatment, and neoadjuvant therapy, breast cancer remains a prominent cause of mortality on a global scale. The tumor microenvironment (TME) is of paramount importance in the context of therapeutic resistance and the advancement of cancer. Hypoxia, characterized by a low level of oxygen, is a prevalent attribute found in the majority of TME. Hypoxia induces the production of reactive oxygen species (ROS), leading to the occurrence of oxidative stress. The occurrence of hypoxia-induced oxidative stress results in the modification of cancer cell metabolism, impaired vascularization, enhanced cell motility, and metastasis, ultimately resulting in the acquisition of epithelial-to-mesenchymal transition (EMT). Hence, the presence of hypoxia-induced oxidative stress poses a substantial obstacle to the successful implementation of cancer therapies. Hypoxia-inducible factor-1α (HIF-1α) plays a pivotal role in the cellular response to hypoxia in tumors. It initiates the transcription of a multitude of genes, encompassing pro-angiogenic and pro-metastatic genes. Another significant signaling molecule that is activated in response to tumor hypoxia and oxidative stress is nuclear factor erythroid 2–related factor 2 (Nrf2). The regulatory role of ROS encompasses the modulation of both HIF-1α and Nrf2 signaling pathways. Moreover, the essentiality of coordinated signaling between HIF-1α and Nrf2 for tumor survival, metastasis, and chemo-resistance is evident, thereby contributing to the progression of tumors. While HIF-1α is widely recognized as a crucial mediator of the cellular reaction to low oxygen levels, it is important to note that Nrf2, a transcription factor responsible for regulating antioxidants, plays a critical role in facilitating HIF-1α-mediated responses to hypoxia. This review article places emphasis on the role of hypoxia-induced oxidative stress in the progression of breast cancer, discussing the underlying mechanisms associated with this phenomenon.
Keywords: Oxidative stress, Hypoxia, Breast cancer, Nrf2, Angiogenesis, Metastasis
Introduction
Cancer
Cancer is characterized by the presence of an anomalous aggregation of cells that have undergone a loss of regulatory mechanisms, resulting in uncontrolled proliferation. It has the potential to manifest in nearly any anatomical organ. In order for it to be detected, the size of the entity must attain a magnitude of 1 cm (~ 1 million cells). In the year 2020, a global total of approximately 19.3 million instances of cancer were documented, resulting in approximately 10 million fatalities [1]. According to a report, the prevalence of cancer patients in India in the year 2020 was documented at 1.39 million cases [2]. Cancer ranks as the second most prevalent cause of death on a global scale, affecting approximately one in four individuals with a lifetime risk. The combination of an unfavorable prognosis, elevated mortality rates, and limited therapeutic options contribute to the substantial impact of this issue on public health [3].
Breast cancer
Breast cancer (BC) is the most prevalent form of cancer and a substantial contributor to mortality associated with cancer in women on a global scale. According to recent data, the year 2020 witnessed a considerable number of women, approximately 2.3 million, receiving a diagnosis of BC worldwide. Tragically, this disease also claimed the lives of approximately 685,000 individuals during the same period [1]. BC accounts for approximately 30% of the total incidence of new cancer cases among women [4]. BC originates within the breast tissue, primarily affecting the milk ducts and lobules. Ductal carcinoma, which accounts for approximately 85% of cases, refers to cancer that originates from the ductal region. On the other hand, the neoplastic growth originating from the mammary lobules is referred to as lobular carcinoma, accounting for approximately 15% of all cases. The presence of a malignant neoplasm limited to either the duct or lobule is typically asymptomatic and exhibits the lowest propensity for metastatic dissemination. Nevertheless, as time elapses, cancer has the potential to advance and infiltrate the adjacent breast tissue, resulting in invasive breast cancer. Furthermore, it can disseminate to the nearby lymph nodes, known as regional metastasis, or to distant organs throughout the body, referred to as distant metastasis. This process, commonly referred to as metastasis, is primarily accountable for treatment ineffectiveness and represents the leading cause of mortality in BC cases [5]. While the majority of cancer types exhibit a higher incidence rate among males compared to females, BC deviates from this pattern, displaying a higher incidence rate among females than males [6]. The prevalence of this condition is significantly higher in women compared to men, with a ratio of 100:1. The incidence of breast cancer in men has exhibited stability over the course of the last three decades, with a lifetime risk of less than 1% [7].
There are multiple factors that contribute to an increased susceptibility to developing BC. The risk factors for BC encompass various factors such as age, obesity, alcohol consumption, radiation exposure, tobacco use, family history of breast cancer, reproductive history (including age at onset of menstruation and first pregnancy), postmenopausal hormone therapy, and mutations in the genes BRCA1, BRCA2, and PALB-2 [8]. In a general sense, symptoms associated with BC include:
- Painless lump (thickening in the breast)
- Variation in appearance, shape, and size of a breast
- Redness, dimpling, or other variation in the skin
- Change in nipple appearance and abnormal nipple discharge [9].
However, in most cases, lumps are not cancerous. Therefore, a complete medical examination, comprising imaging of the breast and biopsy, is necessary to determine if a mass is benign (noncancerous) or malignant (cancerous). BC is a highly complex disease with a high degree of diversity within tumors [10]. Therefore, the prognosis of the BC patient depends on the molecular subtype of cancer. Depending on the presence or absence of the Estrogen Receptor (ER), Progesterone Receptor (PR), and Human Epidermal Growth Factor Receptor (HER2) there are four main BC molecular subtypes [11] as follows:
- Luminal A (ER+/PR+/HER2–)
- Luminal B (ER+/PR+/HER2+)
- Triple-negative (ER–/PR–/HER2–)
- HER2-positive (ER–/PR–/HER2+)
Among all, luminal A (ER+/PR+/HER2–) is the most diagnosed BC subtype with a lower rate of metastasis, while HER2+(ER–/PR–/HER2+) is the least prevalent of the four subtypes [12]. Luminal B (ER+/PR+/HER2+) is positive for all threereceptors. Furthermore, triple-negative breast cancer (TNBC) (ER–/PR–/HER2–) possesses the highest recurrence and metastatic rate with the lowest overall 5-year survival rate [13][14]. The presence of hormone receptors allows for targeted endocrine therapy. HER2+ permits the utilization of targeted anti-HER2 therapeutics to decrease tumor volume and the likelihood of metastasis [15]. However, due to the lack of hormone receptors in TNBC, it lacks predictive markers, and therefore chemotherapy and radiation therapy are the sole options to decrease tumor volume and prevent metastasis [15][16]. TNBC is frequently seen in younger patients, specifically premenopausal women of <40 years of age [17]. TNBC accounts for approximately 15–20% of all BC cases [18]. It possesses characteristics such as increased invasiveness with enhanced metastatic potential, chemo-resistance, elevated rate of relapse, and worse overall prognosis [19][20]. Development of chemo-resistance within the TNBC subtype reduces the postoperative treatment’s efficacy, hence increasing the likelihood of recurrence at a distant site. Therefore, extensive research has been devoted to understanding the mechanisms of adaptation and resistance to chemotherapeutics within TNBC [21]. The anticipated mechanisms for chemo-resistance within TNBC depend on the original tumor’s heterogeneity, the proportion of cancer stem cells present, and the communication of BC cells with other cells in the tumor microenvironment (TME) [22][23]. Recent studies showed that Hypoxia in TME is strongly correlated to clinic-pathological features, and the development of different tumors and BC is among them [24].
Hypoxia
Hypoxia refers to the condition in which tumor cells experience a lack of molecular oxygen. Hypoxia represents a common characteristic of the TME in nearly all types of solid tumors. The phenomenon occurs due to the rapid proliferation of cancer cells, leading to the surpassing of the surrounding vasculature’s capacity. Consequently, this causes a decline in normal oxygen levels (≥40 mmHg) and the eventual attainment of hypoxic levels (0–20 mmHg) [25][26]. In fact, the development of normal tissues is characterized by a synchronized process with the formation of blood vessels, whereas in the context of cancer, tumor growth is facilitated by the activation of oncogenes independent of a supportive vascular network. Hypoxia typically occurs within solid tumors when they are located approximately 100μm away from a functional blood vessel [27]. Based on oxygen concentration, solid tumors contain three regions.
- Normoxic (located near blood vessels). Normoxic cells are typically viable and proliferative
- Hypoxic (peri-necrotic cells with the ability to exist at very low oxygen levels (PO2 ≤1%)
- Necrotic (located 150μm from a parent blood vessel and contains no oxygen) [28].
Hypoxia typically causes cell death in normal cells. However, in tumor cells hypoxia leads to mutations and allows them to adapt to limited nutrition and the harsh TME, enabling their survival. Under these circumstances, selected viable cells release pro-angiogenic factors, stimulating new blood vessel formation and contributing to malignant progression [29][30]. Low oxygen levels impact signaling pathways through HIF-1, leading to drug resistance and a worse prognosis. Hypoxic tumor cells drive tumor progression [31]. Hypoxia is associated with poor clinical outcomes and interferes with successful treatment. HIF is a hypoxia-responsive transcription factor upregulated in response to hypoxia [32]. The HIF family consists of three members: HIF-1 (expressed in tumor cells), HIF-2 (expressed in tumor-associated macrophages), and HIF-3 (expressed in the human kidney and pulmonary alveolar epithelial cells) [33-36].
HIF is composed of α and β subunits, forming a heterodimeric structure. Under normal oxygen levels (normoxic conditions), the α and β subunits of HIF dissociate. HIF-1α is a crucial component in the cellular processes that are activated in response to low oxygen levels [37]. Under normal oxygen levels, the enzymatic activity of prolyl hydroxylases (PHDs) is responsible for the hydroxylation of the oxygen-dependent degradation domain (ODDD) of HIF-1α. Hydroxylated HIF-1α is identified by von Hippel–Lindau (VHL) protein. VHL protein has been observed to recruit an E3 ubiquitin ligase, which is responsible for catalyzing the poly-ubiquitination of HIF-1α. This process ultimately leads to the degradation of HIF-1α through the proteasomal pathway [37]. Under hypoxic conditions, the process of ubiquitination is suppressed for HIF-1α. Consequently, HIF-1α becomes stabilized and associates with the HIF-1β subunit to form an active HIF-1 complex. Active HIF-1 complex induces transcription of target genes containing hypoxia response element (HRE); which is represented by the core sequence 5′-RCGTG-3′ [38]. Thus, HIF1 is known to exert a significant influence on various crucial aspects of tumor biology. These include angiogenesis [39][40], epithelial-to-mesenchymal transition (EMT) [41][42], invasion [43], metastasis [44][45], stem cell maintenance [46], as well as resistance to radiation therapy [47] and chemotherapy [48].
Hypoxia induces the generation of Reactive Oxygen Species (ROS), causing Oxidative Stress. ROS stabilizes the expression of HIF-1α thereby regulating the expression of several genes involved in the modulation of tumor growth and progression [49]. Therefore, a detailed understanding of the role and mechanism of action of hypoxia-mediated oxidative stress in regulating HIF-1α activity and tumor progression will help develop potential therapeutic interventions.
Oxidative stress in breast cancer
A hallmark of hypoxia is the formation of ROS, causing oxidative stress. An imbalance between the generation of ROS and antioxidant defense leads to oxidative stress in the body. Superoxide (O2•−), hydrogen peroxide (H2O2), hydroxyl radicals (•OH), and singlet oxygen (1O2) are known as ROS [50][51]. ROS are by-products of aerobic metabolism, which possesses strong oxidizing ability. Every cell in the human body is exposed to ~1.5×105 oxidative hits per day [52]. At low concentrations, ROS functions as a secondary messenger maintaining cellular homeostasis while they are deleterious to cells at a higher concentration by causing damage to DNA, lipids, and proteins [53]. Although the accumulation of ROS in hypoxic cells is not clearly understood, studies suggest that lack of molecular oxygen under hypoxia blocks the formation of ATP by shutting down the mitochondrial electron transport chain (ETC) [54]. Electrons that would otherwise be funneled into ETC are then transferred to molecular oxygen in an incomplete reduction reaction resulting in the formation of oxygen radicals (superoxide and hydroxyl radicals). These ROS can damage DNA, RNA, act as a signaling molecule activating the pro-survival pathway, including PI3K/MAPK pathway, and thus, contribute to tumor progression [55].
Cancer cells exhibit heightened metabolic activity and experience low oxygen levels, resulting in an increased production of ROS molecules. These molecules have the potential to cause DNA damage and serve as secondary messengers in various redox-sensitive signaling pathways that play crucial roles in cell survival, tumor resistance, and tumor progression [56]. The phenomenon of oxidative stress plays a role in the promotion of cancer stemness, angiogenesis, invasion, and metastatic capacity. Consequently, the regulation of oxidative stress has emerged as a significant approach in the realm of cancer prevention [57]. Cells have a special defense system called ‘antioxidants’ which convert unstable free radicals into stable, less damaging molecules, thus maintaining ROS at physiologically normal levels [58]. In response to ROS, various transcription factors are activated, such as Nrf2, HIF-1α, p53, NF-κB, STAT, and AP-1 [59-65]. Among all transcription factors, Nrf2/Keap1 pathway plays a key role in the regulation of cellular response to oxidative stress.
Nrf2/Keap1 pathway
Nuclear factor erythroid 2-related factor 2 (Nrf2) is an antioxidant transcription factor that binds to and mediates the expression ofantioxidant response element (ARE) containing genes. Nrf2 is complexed with Kelch-like ECH-associated protein 1 (Keap1) in thecytoplasm under normal cellular conditions, maintaining Nrf2 at a low level. However, inresponse to various stresses, Nrf2 is de-repressed and induces the expression of ARE-containing genes.Human Nrf2 is 605 amino acids long which contains six highly conserved domains known asNeh (Nrf2-ECH) domains. Neh1 domain contains a bZIP motif and helps in binding to ARE.Neh2 domain acts as a negative regulatory domain. Neh3 domain helps in Nrf2 transactivation. The Neh4 and Neh5 domains are indispensable for Nrf2 transactivation, and the Neh6 domain is needed for Nrf2 protein degradation [66][67]. On the other hand, Keap1 possesses five discrete domains: NTR (N-terminal region), BTB (Broad complex, Tramtrack, and Bric-à-Brac), IVR (intervening region), DGR (double glycine repeat or Kelch repeats), and CTR (C-terminal region). The DGR and CTR domains of Keap1 form a β-propeller structure (together known as DC domain) and interact with the Neh2 domain of Nrf2. IVR domain of Keap1 comprises 8 cysteine residues (C196, C226, C241, C249, C257, C273, C288 and C297), of which, C273 and C288 act as electrophilic stress sensors while the BTB domain helps to dimerize Keap1 [68].
Under normal cellular conditions, Nrf2 is bound with its actin-binding protein Keap1 in the cytoplasm. Keap1, also known as an inhibitor of Nrf2 (INrf2), exists as a dimer inside the cells. It is a substrate adapter for the E3-ubiquitin ligase (Cul3/Rbx1) complex with Nrf2, causing poly-ubiquitination and proteasomal degradation of Nrf2. However, in response to stress (oxidative stress, electrophilic stress, or chemical inducers), key cysteine sulfhydryl groups in keap1 (especially Cys273 and Cys288) are modified, which leads to dissociation of Nrf2 from keap1. Nrf2 becomes stabilized and translocates into the nucleus. Once inside the nucleus, Nrf2 interacts with its binding partner, especially small musculoaponeurotic fibrosarcoma (sMaf), and induces the expression of ARE-containing genes [69]. Nrf2 mediates the transcription of ARE-containing phase II cytoprotective enzymes including NAD[P]H:quinone oxidoreductase-1 (NQO1), Heme oxygenase-1 (HO-1), γ-glutamyl cysteine ligase (γ-GCS), and glutathione S-transferase (GST) [70][71], which are involved in the removal of intracellular ROS. The expression of Nrf2-dependent antioxidant genes is essential for maintaining cellular redox homeostasis by decreasing oxidative stress and protecting the body from numerous diseases. However, there is a “dark side of Nrf2” [72]. Nrf2 and its downstream genes are overexpressed in various cancers, including BC, giving cancer cells survival and growth advantages [73].
Numerous studies have shown that proliferation rates of various cell lines vary depending on their Nrf2 status. Cells overexpressing Nrf2 proliferate faster than wild-type cells, while cells with Nrf2 knockdown proliferate more slowly [73-75]. NRF2 activation also reduces apoptosis, while its inhibition increases the number of apoptotic cells. Mechanistically, Nrf2 activation increases the expression of B-cell lymphoma 2 (Bcl2) and B-cell lymphoma-extra large (Bcl-xl), reduces caspase-3/7 activation, and reduces the release of cytochrome c release from the mitochondria [76][77]. Activated Nrf2 in cancer cells has been shown to promote angiogenesis [78][79], metastasis [80][81], radioresistance [82], chemoresistance [83], and thereby contribute to tumor progression.
Apart from cytoprotection and chemoresistance, Nrf2-mediated anti-oxidation is essential for HIF-1-mediated hypoxic responses. ROS regulates both HIF-1 and Nrf2 signaling. Numerous studies have shown that the inhibition of Nrf2 significantly reduced the HIF-1 α level [78-84]. Thus, the crosstalk between Nrf2 and HIF-1 is essential for tumor cell survival, angiogenesis, invasion and metastasis, and radio and chemoresistance. Therefore, understanding the role and regulation of Nrf2 in tumor angiogenesis and metastasis is necessary to inhibit tumor progression.
Hypoxia in breast tumor angiogenesis
Angiogenesis and angiogenic signaling
Angiogenesis, derived from the Greek words “angio” meaning blood, and “genesis” meaning formation, refers to the physiological process through which new blood vessels are generated from pre-existing vessels. This process differs from vasculogenesis, which involves the formation of new blood vessels from precursor cells known as endothelial precursor cells or angioblasts. Vasculogenesis occurs mainly during embryonic development, and in adults, it is mainly restricted to the heart, brain, or pathophysiology of endometriosis. Angiogenesis occurs during development, wound healing, the menstrual cycle, and pregnancy as a controlled series of events, supporting the tissue requirements. However, in pathological conditions such as cancer, the same angiogenic signaling pathways are induced and abused for cancer progression. To promote angiogenesis, tumor cells tilt the balance between pro and anti-angiogenic factors towards stimulatory angiogenic signals, a process known as an angiogenic switch. Angiogenesis supports the growth of tumors beyond the size of 1-2 mm3 [85].
The process of angiogenesis encompasses a series of four distinct steps:
- The enzymatic degradation of components within the extracellular matrix (ECM)
- The activation and migration of endothelial cells (ECs)
- The proliferation of endothelial cell
- The transformation of endothelial cells, wherein they assume a tubular morphology and organize themselves into capillary tubes, ultimately gives rise to the formation of unique basement membranes.
During tumor growth and proliferation, different types of regulators are released from tumor cells, endothelial cells, stromal cells, and ECM. Vascular endothelial growth factor (VEGF), basic fibroblast growth factor (bFGF), transforming growth factor-α and -β (TGF-α and -β), epidermal growth factor (EGF), placental-derived growth factor (PlGF), platelet-derived growth factor (PDGF), interleukin (IL)-8, cyclooxygenase-2 (COX-2) and angiopoietins 1 and 2 are some of the well-known pro-angiogenic regulators. Angiostatin, endostatin, tumstatin, IL-12, thrombospondin-1 (TSP-1), interferon-α, -β and –γ, platelet factor-4, and tissue inhibitors of metalloproteinases (TIMPs) are some well-known anti-angiogenic regulators [86-91].
In mature capillaries, the vessel wall is made of EC lining, a basement membrane, and a layer of cells called pericytes. Once tumor volume reaches a few mm3, oxygen and nutrient supply become limiting, and tumor cells undergo an angiogenic switch, resulting in the production and release of growth factors into the surrounding tissue. Angiogenic factors released by tumor cells bind to EC receptors and initiate the process of angiogenesis. Once stimulated, ECs secrete proteases such as matrix metalloproteinases (MMPs), heparanase, and other digestive enzymes that aid in the digestion of the basement membrane surrounding the vessel [92]. The junctions between ECs become altered, and the cell projections grow toward the source of the stimulus. ECs migrate and proliferate into the growth factor gradient, forming new vascular structures in the tumor mass. Finally, matrix proteins are deposited, mature pericytes stabilize the new vessels, and functional blood vessels are formed. The formation and maturation of new blood vessels are driven by interactions between cell surface proteins and the ECM. Some of the surface proteins identified in this interaction are VE-cadherin, galectin-2, hybrid oligosaccharides, and PECAM-1 [93-95].
Different situations can provoke an unbalanced shift toward pro-angiogenic factors and trigger an angiogenic switch. These are:
- Metabolic stress (hypoxia, low pH, or hypoglycemia)
- Genetic mutations (activation of oncogenes or loss of tumor-suppressor)
- Mechanical stress (pressure generated by proliferating cells)
- Immune/inflammatory response [91-96].
Hypoxia is one of the main factors that drive tumor angiogenesis by promoting increased expression, and secretion of VEGF and other pro-angiogenic factors from hypoxic cells [97]. Nrf2 and HIF-1α signaling plays a major role in hypoxia-mediated tumor angiogenesis.
Nrf2 in breast tumor angiogenesis
Nrf2/HO-1 pathway is the master antioxidant regulator in a cell, protecting cells against oxidative damage and thus considered a favorable signaling pathway. Nevertheless, the data unveiled the adverse aspects of this pathway, bestowing upon cancer cells the ability to thrive, fostering the growth of new blood vessels, facilitating the spread of cancer to other parts of the body, and conferring resistance to chemotherapeutic substances. [98]. Nrf2 contributes to tumor angiogenesis via various mechanisms:
- The knockdown of Nrf2 has been shown to reduce angiogenesis and subsequent tumor growth in xenograft mouse models. Mechanistically, the knockdown of Nrf2 reduced the HIF-1α protein levels and thereby decreased the expression of pro-angiogenic factors [78][99].
- Nrf2 downstream gene NQO1 directly binds to the ODDD site of HIF-1α. This binding prevents PHDs from interacting with HIF-1α, thereby inhibiting its proteasomal degradation.
- Nrf2 promotes hypoxia-mediated angiogenesis of cardiac microvascular endothelial cells through HO-1 [100].
- Further studies have shown the role of Nrf2 in follicle-stimulating hormone-induced (FSH-induced) angiogenesis in human epithelial ovarian cancer via VEGF expression [101]. The activated Nrf2 increases the expression of HO-1 and IL-8. HO-1 overexpression, in turn, increases VEGF expression. The IL-8 and VEGF act as pro-angiogenic factors [102][103].
These studies illustrate the complex role of Nrf2 in tumor angiogenesis.
Hypoxia in breast tumor metastasis
Metastasis and epithelial-to-mesenchymal transition
Metastasis is a complex process by which cancer cells spread from primary sites to distant sites of the body. It is a complex and deadly process that has been associated with the distant spread and cancer-associated mortality. The process of metastasis involves several stages as follows:
- Activation of EMT, during which cancer cells lose cell-cell, cell-substrate contact, and acquire cell migration.
- Local invasion (during which malignant cells degrade the basal lamina, the special ECM)
- Intravasation (tumor cells enter the bloodstream)
- Capacity to live in the circulation
- Extravasation (tumor cells exit the bloodstream)
- Distant metastatic colonization (disseminated tumor cells adapt to the new microenvironment and switch from the migratory to the proliferation mode to generate macro metastases) [104].
The location of the metastases is not always accidental. Based on the type of cancer, particular organs and tissues are more prone to metastases than others [105]. BC, for example, tends to metastasize more commonly to the bones and lungs, and this specificity is mainly mediated by soluble chemokines [106] and TGF-β [107]. EMT occurs during embryo development, tissue regeneration, wound healing, and organ fibrosis. It is also associated with tumor metastasis and, generation of tumor stem cells and thus contributes to chemoresistance and tumor progression [108][109]. A significant number of molecular pathways cooperate in the process of EMT, ranging from activation of transcription factors, and reorganization of the cytoskeleton to changes in the expression of miRNAs [110]. Vimentin, an EMT marker, is overexpressed in various cancers, including BC, and its overexpression is associated with enhanced tumor growth, invasion, and poor prognosis [111]. MMPs are zinc-binding metalloproteinases involved in the degradation of ECM components, thereby contributing to tumor cell migration and invasion. There are 23 MMPs present in humans, among which MMP9 and MMP2 play a vital role in cancer invasion and metastasis [112][113].
During the phenomenon of metastasis, cells undergo a transition from a differentiated state to a less differentiated state. In general, when normal cells lose contact with the extracellular matrix (ECM), they undergo apoptosis. In contrast, tumor cells have the ability to detach from the primary tumor through the development of specific mechanisms [114]. During the process of epithelial-to-mesenchymal transition (EMT), the permeability of the epithelium is increased through the downregulation of E-cadherin, a crucial protein involved in cell-cell adhesion. This downregulation is mediated by transcription factors, specifically Slug, Snail, Twist, and ZEB1/2 [115]. EMT is a multifaceted mechanism by which cancer cells are able to suppress their epithelial characteristics and enhance their mesenchymal properties. EMT is a developmental process that has been conserved throughout evolution and facilitates the acquisition of motility and migratory capabilities in cancer cells, enabling their movement away from the original tumor site. Thus, EMT plays a role in the development of cancer and enables cancer cells to acquire metastatic properties, including enhanced mobility, invasion, and resistance to apoptosis.
Nrf2 in breast tumor metastasis
The role of Nrf2 in tumor metastasis is complex. Nrf2 expression is essential for the migration of both normal as well as cancer cells as the knockdown of Nrf2 impairs migration and invasion of a variety of cell lines [116][117]. Previous studies showed that Nrf2 promotes EMT by inhibiting E-cadherin expression by an unknown mechanism [80][81]. Furthermore, Nrf2 inhibition reduces N-cadherin expression through downregulation of NOTCH1, a downstream gene of Nrf2 and a regulator of EMT [118][119]. Also, Nrf2 expression is directly associated with the activation of the RhoA/ROCK pathway [76] and is correlated with the activity of gelatinases (MMP2 and MMP9) [120], all of which are involved in the migration and invasion of tumor cells [76][120]. Nrf2 has been shown to promote anchorage-independent growth by inducing the expression of osteopontin, a protein with an essential role in tumor metastasis [121].
Regulation of Nrf2 in breast cancer
Given the importance of Nrf2 in regulating ROS, HIF-1, tumor angiogenesis, and metastasis, understanding its regulation is of utmost importance to develop potential therapeutic interventions. Numerous factors are involved in regulating Nrf2 protein stability and its nuclear translocation:
Keap1
Keap1 is a negative regulator of Nrf2 and functions as a substrate linker protein. Keap1 is present as a dimeric form within the cytoplasm and plays a role in facilitating the interaction between the Cul3/Rbx1-based E3-ubiquitin ligase complex and Nrf2. Consequently, it facilitates the process of Nrf2 ubiquitination and proteasomal degradation. Hence, under basal conditions, Nrf2 is kept at a low level by Keap1-mediated degradation. However, in response to stress (oxidative or electrophilic stress, or chemical inducers), sulfhydryl groups in Keap1, especially Cys151, Cys273, and Cys288, are modified. Modifying these cysteine residues in Keap1 leads to dissociation and activation of Nrf2. Activated Nrf2 then translocates to the nucleus, interacts with additional protein factors, including small musculoaponeurotic fibrosarcoma (sMaf), and induces the transcription of antioxidant genes [69].
p62 (SQSTM1)
The protein p62, also known as sequestosome-1 (SQSTM1), functions as a positive regulator of the transcription factor Nrf2. This protein is a ubiquitin-binding molecule that engages in competitive binding with Nrf2 for the Keap1 protein. The interaction between p62 and Keap1 results in the degradation of Keap1 and the stabilization of Nrf2. Furthermore, Nrf2 activates p62 gene expression, forming a positive feedback loop [122-124].
Protein kinase C (PKC)
PKC is a positive regulator of Nrf2. It phosphorylates Nrf2 at Ser40, which leads to the detachment of Nrf2 from Keap1 and subsequent activation of Nrf2-dependent antioxidant genes [125].
p21Cip1/WAF1
p21Cip1/WAF1 is a cyclin-dependent kinase (CDK) inhibitor. It stabilizes Nrf2 by disrupting the Nrf2-Keap1 interaction [126].
DJ-1
DJ-1 is Parkinson’s disease protein 7 encoded by the Park7 gene. It stabilizes Nrf2 by disrupting the Nrf2-Keap1 interaction [127].
PALB2 and BRCA1
In addition to the factors mentioned above, other proteins that stabilize Nrf2 by affecting the binding between Nrf2 and Keap1 include partner and localizer of BRCA2 (PALB2) and BRCA1 [128][129].
β-TrCP-Cul1
Beta-transducin repeat-containing protein (β-TrCP) is a component of the β-TrCP-Cul1 E3 ubiquitin ligase. β-TrCP is a negative regulator of Nrf2. Phosphorylation of Nrf2 by glycogen synthase kinase-3beta (GSK-3β) enables Nrf2 to be recognized by β-TrCP that in turn marks Nrf2 for ubiquitination and subsequent degradation [130-132]. On the other hand, phosphorylation of GSK-3β by phosphatidylinositol 3-kinase (PI3K)/Akt pathway inhibits GSK-3β and leads to an increase in the Nrf2 protein stability [130].
Hrd1
3-hydroxy-3-methylglutaryl reductase degradation 1 (Hrd1) is a negative regulator of Nrf2. It is an E3 ligase involved in the degradation of misfolded proteins in the endoplasmic reticulum. It suppresses Nrf2 activity through ubiquitination and degradation by the proteasome [133].
Non-coding RNAs
In addition to the aforementioned factors, Nrf2 is also regulated at the posttranscriptional level by long noncoding RNAs and microRNAs (miRNAs). Because of their short size and stability in addition to their ability to rapidly respond to a variety of stresses and target multiple genes and pathways simultaneously, miRNAs are emerging as promising players in cancer therapeutics including BC [134].
Conclusions and future therapeutic perspective
In the current review, the role and mechanism of hypoxia-mediated oxidative stress in breast tumor angiogenesis and metastasis were discussed. The complexity of NRF2-mediated breast cancer progression was also overviewed. Due to these complexities, drug resistance is a significant problem in successful breast cancer treatment strategies. Resistance can develop after prolonged exposure to chemotherapeutic drugs or can exist inherently in the patient. Tumors rapidly develop resistance after exposure to drugs, leading to most cancer-related deaths. Also, traditional drugs operate by inhibiting one or two protein targets, which is inefficient when considering the multitude of protein targets involved in BC. Therefore, in order to develop novel therapeutic strategies and improve patient survival, miRNA-based therapy may provide a novel approach for the future of cancer therapy.
The advantage of miRNA-based therapeutics over protein therapeutics is that they are short-sized, stable, respond rapidly to various stresses, and simultaneously regulate multiple genes and pathways involved in tumor progression. Also, it is possible to supplement tumor-suppressive miRNAs with synthetic oligonucleotides and alleviate effects caused by oncogenic miRNAs through artificial antagonists. Additionally, miRNAs hold the power to inhibit all targets, including non-druggable targets. Therefore, miRNAs are emerging as promising candidates in breast cancer treatment strategies.
References
1 | Sung H, Ferlay J, Siegel RL, Laversanne M, Soerjomataram I, Jemal A, Bray F. Global cancer statistics 2020: GLOBOCAN estimates of incidence and mortality worldwide for 36 cancers in 185 countries. CA Cancer J. Clin. 2021;71(3):209-49. DOI |
2 | Mathur P, Sathishkumar K, Chaturvedi M, Das P, Sudarshan KL, Santhappan S, Nallasamy V, John A, Narasimhan S, Roselind FS, Icmr-Ncdir-Ncrp Investigator Group. Cancer statistics, 2020: report from national cancer registry programme, India. JCO Glob. Oncol. 2020;6:1063-75. DOI |
3 | Chen W, Zheng R, Baade PD, Zhang S, Zeng H, Bray F, Jemal A, Yu XQ, He J. Cancer statistics in China, 2015. CA Cancer J. Clin. 2016;66(2):115-32. DOI |
4 | Torre LA, Islami F, Siegel RL, Ward EM, Jemal A. Global cancer in women: burden and trends. Cancer Epidemiol. Biomark. Prev. 2017;26(4):444-57. DOI |
5 | Wang L, Zhang S, Wang X. The metabolic mechanisms of breast cancer metastasis. Front. Oncol. 2021;10:602416.. DOI |
6 | Ly D, Forman D, Ferlay J, Brinton LA, Cook MB. An international comparison of male and female breast cancer incidence rates. International journal of cancer. 2013;132(8):1918-26. DOI |
7 | Yalaza M, İnan A, Bozer M. Male breast cancer. Eur. J. Breast Health. 2016;12(1):1. DOI |
8 | Feng Y, Spezia M, Huang S, Yuan C, Zeng Z, Zhang L, Ji X, Liu W, Huang B, Luo W, Liu B. Breast cancer development and progression: Risk factors, cancer stem cells, signaling pathways, genomics, and molecular pathogenesis. Genes Dis. 2018;5(2):77-106. DOI |
9 | Koo MM, von Wagner C, Abel GA, McPhail S, Rubin GP, Lyratzopoulos G. Typical and atypical presenting symptoms of breast cancer and their associations with diagnostic intervals: Evidence from a national audit of cancer diagnosis. J. Cancer Epidemiol. 2017;48:140-6. DOI |
10 | Polyak K. Heterogeneity in breast cancer. J. Clin. Investig. 2011;121(10):3786-8. DOI |
11 | Al-Thoubaity FK. Molecular classification of breast cancer: A retrospective cohort study. Ann. Med. Surg. 2020;49:44-8. DOI |
12 | Fallahpour S, Navaneelan T, De P, Borgo A. Breast cancer survival by molecular subtype: a population-based analysis of cancer registry data. Can. Med. Assoc. J. 2017;5(3):E734-9. DOI |
13 | van Maaren MC, de Munck L, Strobbe LJ, Sonke GS, Westenend PJ, Smidt ML, Poortmans PM, Siesling S. Ten‐year recurrence rates for breast cancer subtypes in the Netherlands: a large population‐based study. Int. J. Cancer. 2019;144(2):263-72. DOI |
14 | Xiao W, Zheng S, Yang A, Zhang X, Zou Y, Tang H, Xie X. Breast cancer subtypes and the risk of distant metastasis at initial diagnosis: a population-based study. Cancer Manag. Res. 2018:5329-38. DOI |
15 | Waks AG, Winer EP. Breast cancer treatment: a review. Jama. 2019;321(3):288-300. DOI |
16 | Medina MA, Oza G, Sharma A, Arriaga LG, Hernández Hernández JM, Rotello VM, Ramirez JT. Triple-negative breast cancer: a review of conventional and advanced therapeutic strategies. Int. J. Environ. Health Res. 2020;17(6):2078. DOI |
17 | Morris GJ, Naidu S, Topham AK, Guiles F, Xu Y, McCue P, Schwartz GF, Park PK, Rosenberg AL, Brill K, Mitchell EP. Differences in breast carcinoma characteristics in newly diagnosed African–American and Caucasian patients: A single‐institution compilation compared with the National Cancer Institute’s Surveillance, Epidemiology, and end results database. Cancer. 2007;110(4):876-84. DOI |
18 | Garrido-Castro AC, Lin NU, Polyak K. Insights into molecular classifications of triple-negative breast cancer: improving patient selection for treatment. Cancer Discov. 2019;9(2):176-98. DOI |
19 | Yin L, Duan JJ, Bian XW, Yu SC. Triple-negative breast cancer molecular subtyping and treatment progress. Breast Cancer Res. 2020;22:1-3. DOI |
20 | Kumar P, Aggarwal R. An overview of triple-negative breast cancer. Arch. Gynecol. Obstet. 2016;293:247-69. DOI |
21 | Nedeljković M, Damjanović A. Mechanisms of chemotherapy resistance in triple-negative breast cancer—how we can rise to the challenge. Cells. 2019;8(9):957. DOI |
22 | O’Reilly EA, Gubbins L, Sharma S, Tully R, Guang MH, Weiner-Gorzel K, McCaffrey J, Harrison M, Furlong F, Kell M, McCann A. The fate of chemoresistance in triple negative breast cancer (TNBC). BBA Clin. 2015;3:257-75. DOI |
23 | Kim C, Gao R, Sei E, Brandt R, Hartman J, Hatschek T, Crosetto N, Foukakis T, Navin NE. Chemoresistance evolution in triple-negative breast cancer delineated by single-cell sequencing. Cell. 2018;173(4):879-93. DOI |
24 | Badowska-Kozakiewicz AM, Budzik MP. Triple-negative breast cancer: expression of hypoxia-inducible factor 1α in triple-negative breast cancer with metastasis to lymph nodes. Breast Cancer Surg. 2018. DOI |
25 | Vaupel P, Kallinowski F, Okunieff P. Blood flow, oxygen and nutrient supply, and metabolic microenvironment of human tumors: a review. Cancer Res. 1989;49(23):6449-65. |
26 | Jing X, Yang F, Shao C, Wei K, Xie M, Shen H, Shu Y. Role of hypoxia in cancer therapy by regulating the tumor microenvironment. Mol. Cancer. 2019;18:1-5. DOI |
27 | Helmlinger G, Yuan F, Dellian M, Jain RK. Interstitial pH and pO2 gradients in solid tumors in vivo: high-resolution measurements reveal a lack of correlation. Nat. Med. 1997;3(2):177-82. DOI |
28 | Hall EJ, Giaccia AJ. Radiobiology for the Radiologist. 2006. |
29 | Vaupel P, Harrison L. Tumor hypoxia: causative factors, compensatory mechanisms, and cellular response. Oncologist. 2004;9(S5):4-9. DOI |
30 | Seo BR, DelNero P, Fischbach C. In vitro models of tumor vessels and matrix: engineering approaches to investigate transport limitations and drug delivery in cancer. Adv. Drug Deliv. Rev. 2014;69:205-16. DOI |
31 | Muz B, de la Puente P, Azab F, Kareem Azab A. The role of hypoxia in cancer progression, angiogenesis, metastasis, and resistance to therapy. Hypoxia. 2015:83-92. DOI |
32 | Schito L, Rey S. Hypoxic pathobiology of breast cancer metastasis. Biochim. Biophys. Acta – Rev Cancer. 2017;1868(1):239-45. DOI |
33 | Robinson PJ, Hack C, Merrill EA, Mattie DR. Mathematical model of HIF-1 alpha pathway, oxygen transport and hypoxia. Bethesda, MD: Henry M. Jackson Foundation For the Advancement of Military Medicine. 2017. |
34 | Talks KL, Turley H, Gatter KC, Maxwell PH, Pugh CW, Ratcliffe PJ, Harris AL. The expression and distribution of the hypoxia-inducible factors HIF-1α and HIF-2α in normal human tissues, cancers, and tumor-associated macrophages. Am. J. Pathol. 2000;157(2):411-21. DOI |
35 | Li QF, Wang XR, Yang YW, Lin H. Hypoxia upregulates hypoxia inducible factor (HIF)-3α expression in lung epithelial cells: characterization and comparison with HIF-1α. Cell Res. 2006;16(6):548-58. DOI |
36 | Yang SL, Wu C, Xiong ZF, Fang X. Progress on hypoxia-inducible factor-3: Its structure, gene regulation and biological function. Mol. Med. Rep. 2015;12(2):2411-6. DOI |
37 | Huang Y, Lin D, Taniguchi CM. Hypoxia inducible factor (HIF) in the tumor microenvironment: friend or foe?. Sci. China Life Sci. 2017;60:1114-24. DOI |
38 | Semenza GL. Regulation of mammalian O2 homeostasis by hypoxia-inducible factor 1. Annu. Rev. Cell Dev. Biol. 1999;15(1):551-78. DOI |
39 | Liao D, Johnson RS. Hypoxia: a key regulator of angiogenesis in cancer. Cancer Metastasis Rev. 2007;26:281-90. DOI |
40 | Lee K, Zhang H, Qian DZ, Rey S, Liu JO, Semenza GL. Acriflavine inhibits HIF-1 dimerization, tumor growth, and vascularization. Proc. Natl. Acad. Sci. 2009;106(42):17910-5. DOI |
41 | Esteban MA, Tran MG, Harten SK, Hill P, Castellanos MC, Chandra A, Raval R, O’brien TS, Maxwell PH. Regulation of E-cadherin expression by VHL and hypoxia-inducible factor. Cancer Res. 2006;66(7):3567-75. DOI |
42 | Tam SY, Wu VW, Law HK. Hypoxia-induced epithelial-mesenchymal transition in cancers: HIF-1α and beyond. Front. Oncol. 2020;10:486. DOI |
43 | Krishnamachary B, Semenza GL. Analysis of Hypoxia‐Inducible Factor 1α Expression and its Effects on Invasion and Metastasis. Meth. Enzymol. 2007;435:347-54. DOI |
44 | Chang J, Erler J. Hypoxia-mediated metastasis. Tumor Microenvironment and Cellular Stress: Signaling, Metabolism, Imaging, and Therapeutic Targets. 2014:55-81. DOI |
45 | Chan DA, Giaccia AJ. Hypoxia, gene expression, and metastasis. Cancer Metastasis Rev. 2007;26:333-9. DOI |
46 | Suda T, Takubo K, Semenza GL. Metabolic regulation of hematopoietic stem cells in the hypoxic niche. Cell Stem Cell. 2011;9(4):298-310. DOI |
47 | Moeller BJ, Richardson RA, Dewhirst MW. Hypoxia and radiotherapy: opportunities for improved outcomes in cancer treatment. Cancer Metastasis Rev. 2007;26:241-8. DOI |
48 | Rohwer N, Cramer T. Hypoxia-mediated drug resistance: novel insights on the functional interaction of HIFs and cell death pathways. Drug Resist. Updat. 2011;14(3):191-201. DOI |
49 | Hielscher A, Gerecht S. Hypoxia and free radicals: role in tumor progression and the use of engineering-based platforms to address these relationships. Free Radic. Biol. Med. 2015;79:281-91. DOI |
50 | Sato H, Shibata M, Shimizu T, Shibata S, Toriumi H, Ebine T, Kuroi T, Iwashita T, Funakubo M, Kayama Y, Akazawa C. Differential cellular localization of antioxidant enzymes in the trigeminal ganglion. Neuroscience. 2013;248:345-58. DOI |
51 | Navarro-Yepes J, Zavala-Flores L, Anandhan A, Wang F, Skotak M, Chandra N, Li M, Pappa A, Martinez-Fong D, Del Razo LM, Quintanilla-Vega B, Franco R. Antioxidant gene therapy against neuronal cell death. Pharmacol. Ther. 2014;142(2):206-30. DOI |
52 | Perillo B, Di Donato M, Pezone A, Di Zazzo E, Giovannelli P, Galasso G, Castoria G, Migliaccio A. ROS in cancer therapy: The bright side of the moon. Exp. Mol. Med. 2020;52(2):192-203. DOI |
53 | Thannickal VJ, Fanburg BL. Reactive oxygen species in cell signaling. Am. J. Physiol. Lung Cell Mol. Physiol. 2000;279(6):L1005-28. DOI |
54 | Tafani M, Sansone L, Limana F, Arcangeli T, De Santis E, Polese M, Fini M, Russo MA. The interplay of reactive oxygen species, hypoxia, inflammation, and sirtuins in cancer initiation and progression. Oxid. Med. Cell. Longev. 2016;2016. DOI |
55 | Brahimi-Horn MC, Chiche J, Pouysségur J. Hypoxia and cancer. J. Mol. Med. 2007;85:1301-7. DOI |
56 | Vera-Ramirez L, Ramirez-Tortosa M, Perez-Lopez P, Granados-Principal S, Battino M, Quiles JL. Long-term effects of systemic cancer treatment on DNA oxidative damage: The potential for targeted therapies. Cancer Lett. 2012;327(1-2):134-41. DOI |
57 | Arfin S, Jha NK, Jha SK, Kesari KK, Ruokolainen J, Roychoudhury S, Rathi B, Kumar D. Oxidative stress in cancer cell metabolism. Antioxidants. 2021;10(5):642. DOI |
58 | Halliwell B. Biochemistry of oxidative stress. Biochem. Soc. Trans. 2007;35(5):1147-50. DOI |
59 | Hahn ME, McArthur AG, Karchner SI, Franks DG, Jenny MJ, Timme-Laragy AR, Stegeman JJ, Woodin BR, Cipriano MJ, Linney E. The transcriptional response to oxidative stress during vertebrate development: effects of tert-butylhydroquinone and 2, 3, 7, 8-tetrachlorodibenzo-p-dioxin. PloS one. 2014;9(11):e113158. DOI |
60 | Jennings P, Limonciel A, Felice L, Leonard MO. An overview of transcriptional regulation in response to toxicological insult. Arch. Toxicol. 2013;87:49-72. DOI |
61 | Martindale JL, Holbrook NJ. Cellular response to oxidative stress: signaling for suicide and survival. J. Cell. Physiol. 2002;192(1):1-5. DOI |
62 | Marinho HS, Real C, Cyrne L, Soares H, Antunes F. Hydrogen peroxide sensing, signaling and regulation of transcription factors. Redox Biol. 2014;2:535-62. DOI |
63 | Kietzmann T, Görlach A. Reactive oxygen species in the control of hypoxia-inducible factor-mediated gene expression. InSeminars in cell & developmental biology. Academic Press. 2005;16(4-5):474-86. DOI |
64 | Linher-Melville K, Singh G. The complex roles of STAT3 and STAT5 in maintaining redox balance: Lessons from STAT-mediated xCT expression in cancer cells. Mol. Cell. Endocrinol. 2017;451:40-52. DOI |
65 | Linher-Melville K, Haftchenary S, Gunning P, Singh G. Signal transducer and activator of transcription 3 and 5 regulate system Xc-and redox balance in human breast cancer cells. Mol. Cell. Biochem. 2015;405:205-21. DOI |
66 | Keum YS. Regulation of the Keap1/Nrf2 system by chemopreventive sulforaphane: implications of posttranslational modifications. Ann. N. Y. Acad. Sci. 2011;1229(1):184-9. DOI |
67 | Taguchi K, Motohashi H, Yamamoto M. Molecular mechanisms of the Keap1–Nrf2 pathway in stress response and cancer evolution. Genes Cells. 2011;16(2):123-40. DOI |
68 | Ogura T, Tong KI, Mio K, Maruyama Y, Kurokawa H, Sato C, Yamamoto M. Keap1 is a forked-stem dimer structure with two large spheres enclosing the intervening, double glycine repeat, and C-terminal domains. Proc. Natl. Acad. Sci. 2010;107(7):2842-7. DOI |
69 | Yamamoto M, Kensler TW, Motohashi H. The KEAP1-NRF2 system: a thiol-based sensor-effector apparatus for maintaining redox homeostasis. Physiol. Rev. 2018;98(3):1169-203. DOI |
70 | Kobayashi A, Kang MI, Watai Y, Tong KI, Shibata T, Uchida K, Yamamoto M. Oxidative and electrophilic stresses activate Nrf2 through inhibition of ubiquitination activity of Keap1. Mol. Cell Biol. 2006;26(1):221-9. DOI |
71 | Nguyen T, Nioi P, Pickett CB. The Nrf2-antioxidant response element signaling pathway and its activation by oxidative stress. J. Biol. Chem. 2009;284(20):13291-5. DOI |
72 | Lau A, Villeneuve NF, Sun Z, Wong PK, Zhang DD. Dual roles of Nrf2 in cancer. Pharmacol. Res. 2008;58(5-6):262-70. DOI |
73 | Homma S, Ishii Y, Morishima Y, Yamadori T, Matsuno Y, Haraguchi N, Kikuchi N, Satoh H, Sakamoto T, Hizawa N, Itoh K. Nrf2 enhances cell proliferation and resistance to anticancer drugs in human lung cancer. Clin. Cancer Res. 2009;15(10):3423-32. DOI |
74 | Lister A, Nedjadi T, Kitteringham NR, Campbell F, Costello E, Lloyd B, Copple IM, Williams S, Owen A, Neoptolemos JP, Goldring CE. Nrf2 is overexpressed in pancreatic cancer: implications for cell proliferation and therapy. Mol. Cancer. 2011;10(1):1-3. DOI |
75 | Zhang C, Wang HJ, Bao QC, Wang L, Guo TK, Chen WL, Xu LL, Zhou HS, Bian JL, Yang YR, Sun HP. NRF2 promotes breast cancer cell proliferation and metastasis by increasing RhoA/ROCK pathway signal transduction. Oncotarget. 2016;7(45):73593. DOI |
76 | Niture SK, Jaiswal AK. Nrf2 protein up-regulates antiapoptotic protein Bcl-2 and prevents cellular apoptosis. J. Biol. Chem. 2012;287(13):9873-86. DOI |
77 | Niture SK, Jaiswal AK. Nrf2-induced antiapoptotic Bcl-xL protein enhances cell survival and drug resistance. Free Radic. Biol. Med. 2013;57:119-31. DOI |
78 | Kim TH, Hur EG, Kang SJ, Kim JA, Thapa D, Lee YM, Ku SK, Jung Y, Kwak MK. NRF2 blockade suppresses colon tumor angiogenesis by inhibiting hypoxia-induced activation of HIF-1α. Cancer Res. 2011;71(6):2260-75. DOI |
79 | Ji X, Wang H, Zhu J, Zhu L, Pan H, Li W, Zhou Y, Cong Z, Yan F, Chen S. Knockdown of Nrf2 suppresses glioblastoma angiogenesis by inhibiting hypoxia‐induced activation of HIF‐1α. Int. J. Cancer. 2014;135(3):574-84. DOI |
80 | Shen H, Yang Y, Xia S, Rao B, Zhang J, Wang J. Blockage of Nrf2 suppresses the migration and invasion of esophageal squamous cell carcinoma cells in hypoxic microenvironment. Dis. Esophagus. 2014;27(7):685-92. DOI |
81 | Arfmann-Knübel S, Struck B, Genrich G, Helm O, Sipos B, Sebens S, Schäfer H. The crosstalk between Nrf2 and TGF-β1 in the epithelial-mesenchymal transition of pancreatic duct epithelial cells. PLoS One. 2015;10(7):e0132978. DOI |
82 | Singh A, Bodas M, Wakabayashi N, Bunz F, Biswal S. Gain of Nrf2 function in non-small-cell lung cancer cells confers radioresistance. Antioxid Redox Signal. 2010;13(11):1627-37. DOI |
83 | Shibata T, Kokubu A, Gotoh M, Ojima H, Ohta T, Yamamoto M, Hirohashi S. Genetic alteration of Keap1 confers constitutive Nrf2 activation and resistance to chemotherapy in gallbladder cancer. Gastroenterology. 2008;135(4):1358-68. DOI |
84 | Lu Y, Wang B, Shi Q, Wang X, Wang D, Zhu L. Brusatol inhibits HIF-1 signaling pathway and suppresses glucose uptake under hypoxic conditions in HCT116 cells. Sci. Rep. 2016;6(1):39123. DOI |
85 | Ribatti D, Nico B, Crivellato E, Roccaro AM, Vacca A. The history of the angiogenic switch concept. Leukemia. 2007;21(1):44-52. DOI |
86 | Ferrara N, Adamis AP. Ten years of anti-vascular endothelial growth factor therapy. Nat. Rev. Drug Discov. 2016;15(6):385-403. DOI |
87 | Mihic-Probst D, Ikenberg K, Tinguely M, Schraml P, Behnke S, Seifert B, Civenni G, Sommer L, Moch H, Dummer R. Tumor cell plasticity and angiogenesis in human melanomas. PloS one. 2012;7(3):e33571. DOI |
88 | Liekens S, Schols D, Hatse S. CXCL12-CXCR4 axis in angiogenesis, metastasis and stem cell mobilization. Curr. Pharm. Des. 2010;16(35):3903-20. DOI |
89 | Bridgeman VL, Vermeulen PB, Foo S, Bilecz A, Daley F, Kostaras E, Nathan MR, Wan E, Frentzas S, Schweiger T, Hegedus B. Vessel co‐option is common in human lung metastases and mediates resistance to anti‐angiogenic therapy in preclinical lung metastasis models. J. Pathol. 2017;241(3):362-74. DOI |
90 | Hlushchuk R, Riesterer O, Baum O, Wood J, Gruber G, Pruschy M, Djonov V. Tumor recovery by angiogenic switch from sprouting to intussusceptive angiogenesis after treatment with PTK787/ZK222584 or ionizing radiation. Am. J. Pathol. 2008;173(4):1173-85. DOI |
91 | Kerbel RS. Tumor angiogenesis: past, present and the near future. Carcinogenesis. 2000;21(3):505-15. DOI |
92 | Bhushan M, Young HS, Brenchley PE, Griffiths CE. Recent advances in cutaneous angiogenesis. Br. J. Dermatol. 2002;147(3):418-25. DOI |
93 | Nangia-Makker P, Honjo Y, Sarvis R, Akahani S, Hogan V, Pienta KJ, Raz A. Galectin-3 induces endothelial cell morphogenesis and angiogenesis. Am. J. Pathol. 2000;156(3):899-909. DOI |
94 | Gamble J, Meyer G, Noack L, Furze J, Matthias L, Kovach N, Harlan J, Vadas M. β1 integrin activation inhibits in vitro tube formation: effects on cell migration, vacuole coalescence and lumen formation. Endothelium. 1999;7(1):23-34. DOI |
95 | Yang S, Graham J, Kahn JW, Schwartz EA, Gerritsen ME. Functional roles for PECAM-1 (CD31) and VE-Cadherin (CD144. in tube assembly and lumen formation in Three-Dimensional collagen gels. Am. J. Pathol. 1999;155(3):887-95. DOI |
96 | Carmeliet P. Controlling the cellular brakes. Nature. 1999;401(6754):657-8. DOI |
97 | Dor Y, Porat R, Keshet E. Vascular endothelial growth factor and vascular adjustments to perturbations in oxygen homeostasis. Am. J. Physiol. Cell Physiol. 2001;280(6):C1367-74. DOI |
98 | Lau A, Wang XJ, Zhao F, Villeneuve NF, Wu T, Jiang T, Sun Z, White E, Zhang DD. A noncanonical mechanism of Nrf2 activation by autophagy deficiency: direct interaction between Keap1 and p62. Mol. Cell Biol. 2010;30(13):3275-85. DOI |
99 | Li L, Pan H, Wang H, Li X, Bu X, Wang Q, Gao Y, Wen G, Zhou Y, Cong Z, Yang Y. Interplay between VEGF and Nrf2 regulates angiogenesis due to intracranial venous hypertension. Sci. Rep. 2016;6(1):37338. DOI |
100 | Kuang L, Feng J, He G, Jing T. Knockdown of Nrf2 inhibits the angiogenesis of rat cardiac micro-vascular endothelial cells under hypoxic conditions. Int. J. Biol. Sci. 2013;9(7):656. DOI |
101 | Zhang Z, Wang Q, Ma J, Yi X, Zhu Y, Xi X, Feng Y, Jin Z. Reactive oxygen species regulate FSH-induced expression of vascular endothelial growth factor via Nrf2 and HIF1α signaling in human epithelial ovarian cancer. Oncol. Rep. 2013;29(4):1429-34. DOI |
102 | Zhang X, Chen X, Song H, Chen HZ, Rovin BH. Activation of the Nrf2/antioxidant response pathway increases IL‐8 expression. Eur. J. Immunol. 2005;35(11):3258-67. DOI |
103 | Zhou S, Ye W, Zhang M. The effects of nrf2 on tumor angiogenesis: a review of the possible mechanisms of action. Crit. Rev. Eukaryot. Gene Expr. 2012;22(2). DOI |
104 | Yeung KT, Yang J. Epithelial–mesenchymal transition in tumor metastasis. Mol. Oncol. 2017;11(1):28-39. DOI |
105 | Nguyen DX, Massagué J. Genetic determinants of cancer metastasis. Nat. Rev. Genet. 2007;8(5):341-52. DOI |
106 | Krol J, Loedige I, Filipowicz W. The widespread regulation of microRNA biogenesis, function and decay. Nat. Rev. Genet. 2010;11(9):597-610. DOI |
107 | Drabsch Y, Ten Dijke P. TGF-β signaling in breast cancer cell invasion and bone metastasis. J Mammary Gland Biol Neoplasia. 2011;16(2):97-108. DOI |
108 | Lambert AW, Pattabiraman DR, Weinberg RA. Emerging biological principles of metastasis. Cell. 2017;168(4):670-91. DOI |
109 | Moustakas A, de Herreros AG. Epithelial–mesenchymal transition in cancer. Mol. Oncol. 2017;11(7):715. DOI |
110 | Bullock MD, Sayan AE, Packham GK, Mirnezami AH. MicroRNAs: critical regulators of epithelial to mesenchymal (EMT) and mesenchymal to epithelial transition (MET) in cancer progression. Biol. Cell. 2012;104(1):3-12. DOI |
111 | Arun S, Shulin L. Vimentin as a potential molecular target in cancer therapy Or Vimentin, an overview and its potential as a molecular target for cancer therapy. Cell. Mol. Life Sci. 2011;68(18):3033-46. DOI |
112 | McCawley LJ, Matrisian LM. Matrix metalloproteinases: they’re not just for matrix anymore!. Curr. Opin. Cell Biol. 2001;13(5):534-40. DOI |
113 | Nagase H, Visse R, Murphy G. Structure and function of matrix metalloproteinases and TIMPs. Cardiovasc. Res. 2006;69(3):562-73. DOI |
114 | Guan X. Cancer metastases: challenges and opportunities. Acta Pharm. Sin. B. 2015;5(5):402-18. DOI |
115 | Thiery JP, Acloque H, Huang RY, Nieto MA. Epithelial-mesenchymal transitions in development and disease. Cell. 2009;139(5):871-90. DOI |
116 | Long M, Rojo de la Vega M, Wen Q, Bharara M, Jiang T, Zhang R, Zhou S, Wong PK, Wondrak GT, Zheng H, Zhang DD. An essential role of NRF2 in diabetic wound healing. Diabetes. 2016;65(3):780-93. DOI |
117 | Li ML, Wang XF, Tan ZJ, Dong P, Gu J, Lu JH, Wu XS, Zhang L, Ding QC, Wu WG, Rao LH. Ethyl pyruvate administration suppresses growth and invasion of gallbladder cancer cells via downregulation of HMGB1-RAGE axis. Int J Immunopathol Pharmacol. 2012;25(4):955-65. DOI |
118 | Wakabayashi N, Shin S, Slocum SL, Agoston ES, Wakabayashi J, Kwak MK, Misra V, Biswal S, Yamamoto M, Kensler TW. Regulation of notch1 signaling by nrf2: implications for tissue regeneration. Sci. Signal. 2010;3(130):ra52-. DOI |
119 | Zhao Q, Mao A, Guo R, Zhang L, Yan J, Sun C, Tang J, Ye Y, Zhang Y, Zhang H. Suppression of radiation-induced migration of non-small cell lung cancer through inhibition of Nrf2-Notch Axis. Oncotarget. 2017;8(22):36603. DOI |
120 | Pan H, Wang H, Zhu L, Mao L, Qiao L, Su X. The role of Nrf2 in migration and invasion of human glioma cell U251. World Neurosurg. 2013;80(3-4):363-70. DOI |
121 | Wagner PJ, Park HR, Wang Z, Kirchner R, Wei Y, Su L, Stanfield K, Guilarte TR, Wright RO, Christiani DC, Lu Q. In vitro effects of lead on gene expression in neural stem cells and associations between up-regulated genes and cognitive scores in children. Environ. Health Perspect. 2017;125(4):721-9. DOI |
122 | Komatsu M, Kurokawa H, Waguri S, Taguchi K, Kobayashi A, Ichimura Y, Sou YS, Ueno I, Sakamoto A, Tong KI, Kim M. The selective autophagy substrate p62 activates the stress responsive transcription factor Nrf2 through inactivation of Keap1. Nat. Cell Biol. 2010;12(3):213-23. DOI |
123 | Jain A, Lamark T, Sjøttem E, Larsen KB, Awuh JA, Øvervatn A, McMahon M, Hayes JD, Johansen T. p62/SQSTM1 is a target gene for transcription factor NRF2 and creates a positive feedback loop by inducing antioxidant response element-driven gene transcription. J. Biol. Chem. 2010;285(29):22576-91. DOI |
124 | Towers CG, Fitzwalter BE, Regan D, Goodspeed A, Morgan MJ, Liu CW, Gustafson DL, Thorburn A. Cancer cells upregulate NRF2 signaling to adapt to autophagy inhibition. Dev. Cell. 2019;50(6):690-703. DOI |
125 | Huang HC, Nguyen T, Pickett CB. Phosphorylation of Nrf2 at Ser-40 by protein kinase C regulates antioxidant response element-mediated transcription. J. Biol. Chem. 2002;277(45):42769-74. DOI |
126 | Chen W, Sun Z, Wang XJ, Jiang T, Huang Z, Fang D, Zhang DD. Direct interaction between Nrf2 and p21Cip1/WAF1 upregulates the Nrf2-mediated antioxidant response. Mol. Cell. 2009;34(6):663-73. DOI |
127 | Clements CM, McNally RS, Conti BJ, Mak TW, Ting JP. DJ-1, a cancer-and Parkinson’s disease-associated protein, stabilizes the antioxidant transcriptional master regulator Nrf2. Proc. Natl. Acad. Sci. 2006;103(41):15091-6. DOI |
128 | Ma J, Cai H, Wu T, Sobhian B, Huo Y, Alcivar A, Mehta M, Cheung KL, Ganesan S, Kong AN, Zhang DD. PALB2 interacts with KEAP1 to promote NRF2 nuclear accumulation and function. Mol. Cell Biol. 2012;32(8):1506-17. DOI |
129 | Gorrini C, Baniasadi PS, Harris IS, Silvester J, Inoue S, Snow B, Joshi PA, Wakeham A, Molyneux SD, Martin B, Bouwman P. BRCA1 interacts with Nrf2 to regulate antioxidant signaling and cell survival. J. Exp. Med. 2013;210(8):1529-44. DOI |
130 | Salazar M, Rojo AI, Velasco D, de Sagarra RM, Cuadrado A. Glycogen synthase kinase-3β inhibits the xenobiotic and antioxidant cell response by direct phosphorylation and nuclear exclusion of the transcription factor Nrf2. J. Biol. Chem. 2006;281(21):14841-51. DOI |
131 | Rada P, Rojo AI, Chowdhry S, McMahon M, Hayes JD, Cuadrado A. SCF/β-TrCP promotes glycogen synthase kinase 3-dependent degradation of the Nrf2 transcription factor in a Keap1-independent manner. Mol. Cell Biol. 2011;31(6):1121-33. DOI |
132 | Rada P, Rojo AI, Evrard-Todeschi N, Innamorato NG, Cotte A, Jaworski T, Tobón-Velasco JC, Devijver H, García-Mayoral MF, Van Leuven F, Hayes JD. Structural and functional characterization of Nrf2 degradation by the glycogen synthase kinase 3/β-TrCP axis. Mol. Cell Biol. 2012;32(17):3486-99. DOI |
133 | Wu T, Zhao F, Gao B, Tan C, Yagishita N, Nakajima T, Wong PK, Chapman E, Fang D, Zhang DD. Hrd1 suppresses Nrf2-mediated cellular protection during liver cirrhosis. Genes Dev. 2014;28(7):708-22. DOI |
134 | Mahajan M, Sitasawad S. Mir-140-5p attenuates hypoxia-induced breast cancer progression by targeting nrf2/ho-1 axis in a keap1-independent mechanism. Cells. 2021;11(1):12. DOI |
Cite this article:
Mahajan, M. An overview of hypoxia-induced oxidative stress and NRF2 role in breast cancer progression. DYSONA – Life Science, 2023;4(2): 50-65. doi: 10.30493/dls.2023.413087