Safir U. Khan 1*; Munir U. Khan 2
1, School of Life Sciences, University of Science and Technology of China, Hefei, China
2, Department of Polymer Science and Engineering, Zhejiang University, Hangzhou, China
E-mail:
safir@mail.ustc.edu.cn
Received: 22/01/2022
Acceptance: 15/02/2022
Available Online: 16/02/2022
Published: 01/04/2022
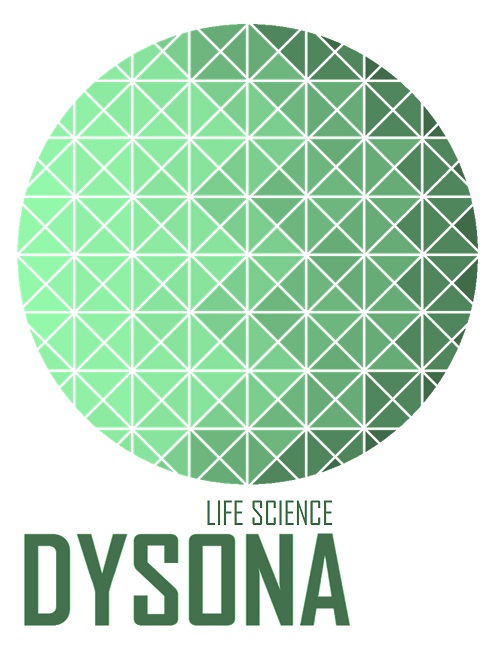
Manuscript link
http://dx.doi.org/10.30493/DLS.2022.325915
Abstract
Fatty liver is the second most widespread liver disease after viral hepatitis. The incidence of fatty liver is increasing, and the age of fatty liver is getting younger, which is a severe threat to human health. The fatty liver consists of a series of pathological symptoms like cirrhosis and hepatocellular carcinoma that may lead to fatty hepatitis as the disease progresses. Generally speaking, fatty liver belongs to reversible disease, through early diagnosis and timely treatment often can return to normal. Therefore, it is critical to understand the pathophysiology of fatty liver disease through cell models. In this review article, the three most commonly used cell models types(primary hepatocytes, immortal cells lines, hepatocyte-like cells from stem cells, and precision-cut liver slices), the three cell culture modes (monoculture, co-culture, and three-dimensional culture), and modeling method (high-Fat, high-sugar, and alcohol) have been summarized and analyzed. It will serve as a reference for fatty liver disease research and drug screening.
Keywords: Non-alcoholic fatty liver disease, Alcoholic fatty liver disease, Cell lines, Cell model
Introduction
Fatty liver is characterized by excessive fat deposition in the liver and clinical manifestations of liver cell inflammation and liver fibrosis. Fatty liver includes non-alcoholic fatty liver disease (NAFLD) and alcoholic fatty liver disease (AFLD). An important indicator to distinguish NAFLD from AFLD is the patient’s drinking situation. AFLD is fatty liver caused by excessive drinking. On the other hand, NAFLD is a disease related to metabolic syndrome and insulin resistance, excluding excessive alcohol and other specific liver injury factors. Its main feature is the excessive deposition of fat in liver cells [1].
At present, the prevalence of fatty liver is increasing globally, and the incidence of NAFLD in Asia is as high as 29.62% [2], which is a severe threat to public health and should be paid attention to by the public. The methods used to study fatty liver include animal models and cell models. The animal model can provide a proper in vivo simulation but is faced with many obstacles, such as the long experiment period and the significant individual differences. For this purpose, the cell model can overcome personal differences and is more straightforward and efficient, which is suitable for high-throughput drug screening and mechanism research. According to the characteristics of fatty liver, researchers at home and abroad have established a variety of cell models, such as high-sugar, high fat and alcohol-induced cells, detection of glucose and lipid metabolism of cells, the establishment of fatty liver cell model, and then screen out functional food factors that can improve fatty liver. The following will be reviewed from cell type selection, cell culture methods, and intervention conditions to reference screening drugs and natural active ingredients.
Fatty liver pathogenesis
Pathogenesis of NAFLD
Recent studies have found that NAFLD is caused by overweight, insulin resistance, lack of exercise, high-fat and high-sugar diet pattern, genetic factors, and intestinal microflora disorder [3](Fig. 1). The main feature of NAFLD is the abnormal accumulation of lipids in the liver. In the absence of inflammation and liver damage, NAFLD is called steatosis or simple fatty liver disease. NAFLD includes simple fatty liver disease, non-alcoholic steatohepatitis, and cirrhosis due to non-alcoholic steatohepatitis. At present, the pathogenesis of NAFLD is still unclear, and the “second strike” theory mentioned most often [4] is explained as follows: First, due to various reasons, the body appears insulin resistance and glucose and lipid metabolism disorders, resulting in the accumulation of a large number of lipids in liver cells, forming NAFLD, which is the “first strike” to the liver. Subsequently, the oxidation of lipids within the cells produces reactive oxygen species (ROS), which causes oxidative stress and inflammatory response, and damage to liver cells, resulting in non-alcoholic steatohepatitis. NASH), the “second strike.” The liver is an essential organ for glucose and lipid metabolism. After the human body ingests food, digestive enzymes decompose starch into monosaccharides into the blood, raising blood glucose and promoting insulin secretion. Insulin regulates blood glucose by (1) promoting the absorption and utilization of glucose in peripheral tissues; (2) promoting the use of glucose in the liver to produce liver glycogen; (3) Promoting adipogenesis and inhibiting the lipolysis of adipose tissue. When insulin resistance occurs in the body, the glucose and lipid metabolism of the body will be disorganized, resulting in fatty liver and diabetes [5].
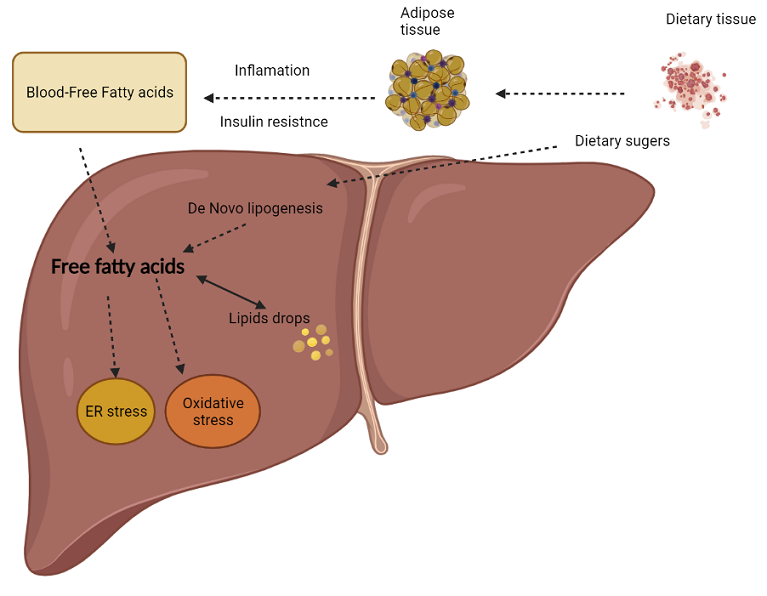
There are two primary sources of liver fat in NAFLD patients.
(1) The free fatty acids decomposed by triglyceride in fat tissue are transported to the liver by blood. Insulin resistance is an essential link in this process. Insulin resistance causes maladjustment of lipolysis in adipose tissue, and excessive fatty acids are transported to the liver, resulting in hepatic steatosis [6].
(2) The de novo synthesis of fatty acids. A high intake of fructose and glucose increases the de novo synthesis of lipids in the liver. Studies have shown that human intestinal epithelium has a limited absorption capacity of fructose, so most fructose is phosphorylated to form fatty acids in the liver. This phosphorylation process leads to the depletion of ATP in the body’s liver, thus increasing the cellular stress response [7].
It has been found that lipid accumulation in Kupffercells can promote the release of inflammatory cytokines interleukin-1 β and tumor necrosis factor-α (TNF-α), leading to the inflammatory response of liver cells [8]. Inflammation can also interfere with insulin signals, leading to insulin resistance [9]. Excessive accumulation of fatty acids in cells can destroy the fluidity of the mitochondrial membrane. This destruction leads to endoplasmic reticulum stress and mitochondrial dysfunction, which subsequently leads to excessive production of reactive oxygen species and ultimately to the occurrence of cellular inflammation and apoptosis, resulting in Sterol regulatory element-binding protein (SREBP). This protein regulates the synthesis of cholesterol, fatty acids, and triglycerides (TG) in the liver and other tissues [10]. Down-regulation of SREBP-1C expression may be beneficial for the treatment of steatohepatitis. Peroxisome proliferators Activated Receptors (PPARs) regulate energy balance and lipid metabolism to transfer fatty acids and carbohydrates from the liver to adipose tissue and enhance fat storage in adipocytes. To reduce the burden of the liver [11], the deregulation of intestinal flora can reduce choline level and increase methylamine level, thereby increasing lipoprotein lipase activity, TG accumulation, and promoting [12].
Pathogenesis of AFLD
The significant risk factors for AFLD include alcohol consumption, obesity, gender, and genetics. Different individuals genetically determine the enzyme activity involved in ethanol metabolism, and different people have strong or weak abilities to metabolize alcohol. The first metabolism and alcohol dehydrogenase activity in women’s stomachs is lower, which increases the risk of disease [13]. Obesity aggravates liver lipid oxidation and accelerates the process of fibrosis and cirrhosis in alcoholic liver disease [14] (Fig. 2).
Alcohol metabolism is mainly carried out in the liver. Ethanol is converted into acetaldehyde, which is highly toxic to the human body, by ethanol dehydrogenase, and then converted into relatively non-toxic acetic acid, which enters the tricarboxylic acid cycle metabolizes into carbon dioxide and water [15]. The following two pathways can induce Alcohol-induced AFLD: (1) Alcohol oxidizes in the liver to produce ROS, which can promote lipid peroxidation and change the permeability of the phospholipid membrane, leading to oxidative stress damage in the liver, leading to liver cirrhosis and other diseases. (2) Alcohol extract is preliminarily metabolized in the liver to produce acetaldehyde, which is highly toxic and can promote the development of AFLD. Acetaldehyde can directly destroy the function of PPAR-α, making free fatty acids unable to be oxidized, transported, and exported, resulting in the accumulation of fatty acids in the liver and the destruction of lipid homeostasis [16]. Acetaldehyde can also damage mitochondria, inhibit the tricarboxylic acid cycle, and damage liver microtubules, thereby affecting lipid metabolism, resulting in lipid deposition in liver cells and the development of fatty liver [17]. In addition, recent studies have found that ethanol can up-regulate ghrelin levels by inhibiting insulin secretion, thus increasing lipid in liver tissues [18].
Studies have found that AFLD is closely related to Toll-like receptor 4(TLR4). Excessive alcohol consumption can increase intestinal permeability, transfer lipopolysaccharide produced by bacteria from the intestinal tract to the liver, activate Kupffer cells, up-regulate the expression of nuclear factor -κB and promote the secretion of TNF-α through lipopolysaccharide /TLR4 pathway [19]. Scientists found that knocking out the TLR4 gene can significantly reduce aspartate aminotransferase (AST) level, TG content, and endogenous adipogenic gene expression [20]. The expression of fatty acid oxidation-related genes increased, suggesting that TLR4 knockdown can improve alcoholic liver injury and fatty liver.
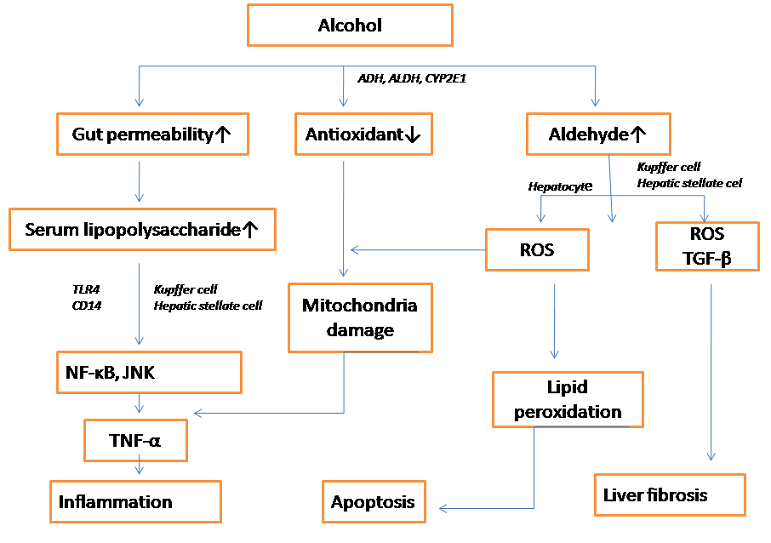
Cell model for studying fatty liver
Liver cells can be divided into two main groups. Liver parenchymal cells, namely normal hepatocytes, and bile duct cells, account for 70% and 3%~5% of the total hepatocytes, respectively, and constitute the main matrix of the liver. The non-parenchymal cells mainly include endodermal cells, Kupffer cells, and stellate cells, accounting for about 25% of the total hepatocytes. Existing model cells can be divided into Human primary hepatocytes, animal primary hepatocytes, immortal cell lines, hepatocyte-like cells (HLCs) derived from induced pluripotent stem cells (iPSCs), and liver sections (Table 1); Culture forms can be divided into a single culture, co-culture and three-dimensional culture (Table 2).
Cell lines used to establish cell models
Human primary hepatocytes
Human primary hepatocytes retain the functions of cells in vivo organs to a large extent, and their metabolic functions and enzyme activities are very similar to those of liver cells in vivo, which is a standard in vitro short-term culture model most close to clinical practice. Human primary hepatocytes are usually obtained from the liver or liver tissue, usually during hepatectomy or liver tissue rejection after liver transplantation, and then immediately studied or frozen for future use. In 1976 [21] proposed the two-step collagenase perfusion technique to separate primary human liver cells. The first step was to remove residual blood in liver tissue and heat the tissue to promote the consumption of calcium ions and ethylene glycol tetraacetic acid and loosen the connection between cells. The second step is to use collagenase infusion to digest the tissue into individual cells, and centrifugal cells can separate the liver cells from the non-parenchymal cells. [22] induced human primary hepatocytes with oleic acid (OA) and palmitic acid (PA) models, showing that intracellular lipid significantly increased and had dose-dependent effects with OA and PA. Lipid accumulation led to TGF-β up-regulation. TGF-β may promote liver fibrosis.
Due to the individual differences of different donors and the influence of various factors in cell separation, the experimental results may be unstable and have poor repeatability. In addition, the culture conditions of human primary hepatocytes are insensitive, which can only be cultured in a short time and cannot be passed on indefinitely, which is not conducive to the development of experiments. The scarcity of human liver samples and ethical and moral issues also hinder the widespread use of human primary liver cells.
Animal primary hepatocytes
Due to the scarcity of human primary hepatocytes and ethical issues, researchers attempted to isolate primary hepatocytes from animal livers for in vitro culture and research. Rodents are more commonly used, along with some mammals and fish. Jia took cow liver cells isolated and cultured by improved two-step irrigation method as the research object, induced them with 0~1 mmol/L sodium oleate for 12 h, and finally selected 0.25 mmol/L as the optimal induction concentration according to cell activity and lipid droplet size [23]. The results showed that the mitochondria vacuolated seriously, and the glycogen particles were less in the treated cells under the electron microscope, which was consistent with the pathological phenomenon of clinical fatty liver. Compared with the control group, the expression level of the PPAR-α gene was significantly increased, and the expression level of lipid synthesis genes SREBP-1C and ChREBP(responsive element-binding protein) was significantly decreased. [24] isolated mouse primary hepatocytes using a two-step collagenase infusion method and cultured them in vitro for a specific time, inducing them with PA at a concentration of 0.2 mmol/L for 24 h. Compared with the control group, cells in the model group had significantly increased intracellular lipids and significantly reduced glucose uptake capacity. The secretion of interleukin-6, interleukin-1 β, and TNF-α was significantly increased in pro-inflammatory cells, and insulin resistance was reduced by regulating the PI3K/ Akt/GSK3β pathway after intervention with Leonuri tricyclic acid.
Animal primary cells solve the ethical problems and the limited number of human primary hepatocytes, but their disadvantages are being affected by different animal sources and batches, poor repeatability, relatively sensitive, and harsh culture conditions.
Immortal cell Lines
Immortal cell lines can be passed on indefinitely and are commonly used by overexpression of viral oncogenes and human telomerase reverse transcriptional protein genes in primary human hepatocytes. Compared with primary hepatocytes, permanent cell lines have the following advantages: stable growth, unlimited longevity, stable phenotype, and more spartan culture conditions than primary hepatocytes, which can be easily standardized between different laboratories. The standard immortal cell lines are human hepatocellular carcinoma cell line HepG2, human standard hepatocellular carcinoma cell line L02, human hepatocellular carcinoma cell line HuH7, human hepatocellular carcinoma cell line HepaRG, and human hepatic stellate cell line LX2.
In another study [25], HepG2 cells were induced with fatty acids for 24 h. The same study reported a significant increase in lipid accumulation, TG, Lactate dehydrogenase (LDH), and malondialdehyde (MALondialdehyde) in cells by oil red O staining. The content of MDA increased significantly, suggesting cell damage and lipid peroxidation. Superoxide dismutase and glutathione were significantly reduced, indicating decreased antioxidant activity. L02 cells were incubated with 3 percent ethanol for 48 hours, which resulted in a considerable increase in AST and Alanine aminotransferase levels (ALANine aminotransferase), TG content, and ROS level, demonstrating that the cells had acquired the characteristics of fatty liver and oxidative stress [26]. HepG2 cells were incubated for 24 hours with 0.5 mmol/L OA, which resulted in elevated TG and ROS levels. Moreover, the ability of cells to absorb glucose decreased significantly, indicating that cells showed symptoms of lipid degeneration, oxidative stress, and insulin resistance [27]. H7 cells were incubated in PA for 24 hours to induce steatosis [28]. They gave HepaRG cells one week of OA to promote steatosis [29]. It was found that when hepatic cells were co-cultured with LX2 in the presence of PA and OA, hepatic cells and hepatocytes became fat-laden [30].
Viral oncogene-mediated immortal cell lines have the risk of tumorigenesis and viral infection. Overexpression of the human telomerase reverse transcription protein is only applicable to human fetal hepatocytes and neonatal hepatocytes but cannot induce the immortalization of adult hepatocytes. In addition, immortalization may damage the chromosomes of cells, resulting in the loss of corresponding functions of cells [31].
Induced pluripotent stem cells-derived hepatocellular-like cells
Induced pluripotent stem cells can differentiate into various somatic cells without causing immune rejection and ethical issues. The morphology and functional characteristics of HLCs derived from human iPSCs are comparable to those of primary hepatocytes. They have the advantages of vast sources, a large number of cultures, and stable phenotype, which make up for the deficiency of primary hepatocytes [32].
Human iPSCs were induced to differentiate into HLCs, and on the 12th day of cell differentiation, all the cell indicators were in line with the characteristics of liver cells [33]. After 50 mmol/L OA was added for 48 h, intracellular lipid accumulation and PLIN2 expression increased, indicating that the cells had, as reported by NAFLD. In another report, pluripotent stem cells were differentiated into HLCs and induced with OA at a concentration of 25 µmol/L and PA at a synergic concentration of 50 µmol/L, 100 µmol/L, and 200 µmol/L for 18 h respectively, then stained with boron-pyrrolidine [34]. The number of lipid droplets was observed to increase with the increase of PA concentration. It is known that TG can induce endoplasmic reticulum stress, and then cells were induced by TG combined with fatty acids to verify whether Endoplasmic reticulum stress can increase the accumulation of exogenous fatty acids in cells. The results showed that endoplasmic reticulum stress could significantly increase intracellular lipid accumulation. However, induced pluripotent hepatocyte technology still has some problems, such as low efficiency of inducing cell transformation, genetic variation in the induction process, and tumorigenicity risk, limiting its wide application.
Precision liver section
The precision liver section is an in vitro culture technology between organ and cell levels. Compared with the cell model, the liver section is closer to the complex structure and composition of the human liver. Liver slices contain liver cells, Kupffer cells, and hepatic stellate cells, creating a multi-cell environment. The interaction of various liver cells can better simulate the environment of liver tissue, but its disadvantage is that it has a short survival time and cannot be cultured for a long time. The preparation method of the precision liver section is to cut the liver tissue of experimental animals with a diameter of about 9 mm in a low-temperature aseptic environment and then put the liver tissue into buffer solution and cut it into slices 200~500 μm with a tissue slicer. Too thick slices will affect the tissue permeability, which is not conducive to the diffusion of oxygen and nutrients. If the section is too thin, the proportion of damaged cells is too large, which affects the experimental results. The liver tissue can be cleaned with a low-temperature buffer solution before sectioning, or the sections can be placed in buffer solution.
The cell viability was higher after cryopreservation for one hour. There are two central culture systems for slice: dynamic tissue culture and continuous immersion tissue culture systems. The former was intermittently infused with O2 and CO2 in a scintillation bottle, and the water bath oscillated at 37 °C. The latter is an oscillating water bath at 37 °C with continuous O2 and CO2 penetration in a porous plate. There was no significant difference between the two culture systems in a short time, and porous plates were used more often due to their low price [35].
The consumption of glutathione, lipid peroxidation, and interleukin-6 in the AFLD liver section model was significantly increased after 24 h of ethanol was added to the culture medium [36]. After 48 h of culture, actin and collagen 1α of smooth muscle increased significantly, suggesting that liver fibrosis was aggravated. Therefore, this model can be used to study AFLD and alcoholic liver injury. Rat liver segment models with a thickness of 250-300 μm were constructed by PRINS and colleagues [37] in order to mimic the metabolic syndrome in humans. The medium was supplemented with 25 mmol/L glucose, five mmol/L fructose, one nmol/L insulin, and 240 mol/L PA. After incubation for 24 h, oil-red O staining and kit detection showed that: With or without PA, lipid droplets increased significantly after co-incubation with glucose, fructose, and insulin. The up-regulation of acetyl-CoA carboxylase 1, acetyl-CoA carboxylase 2, and SrepP-1C suggested an increase in de novo fat formation in liver cells. In addition, the expression of carnitine palmityl transferase 1 decreased, suggesting impaired fatty acid transport and mitochondrial β oxidation disorder. This new in vitro NAFLD model successfully induced steatosis.
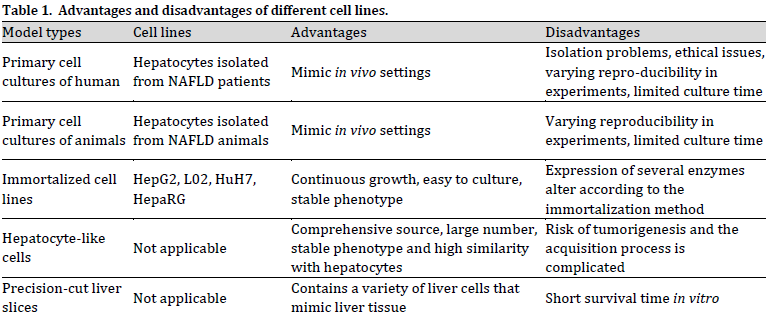
Methods of cell culture
Separate culture Model
Separate culture refers to a cell adherent culture, the most basic culture method. Both primary cells and immortal cells can be cultured separately. The advantages of single culture technology are short cycle, high efficiency, and beneficial to high-throughput drug screening experiments, so it is widely used in the study of fatty liver.
By employing the NAFLD cell model, researchers found that various polyphenols and alkaloid berberine positively affected NAFLD [38]. In the model, HepG2 cells were induced by OA at a concentration of 1.5 mmol/L for 24 h to induce steatosis and cell morphological changes. Subsequently, NAFLD cells were dry-pretreated with polyphenols to detect the expression of genes related to lipid metabolism. The results showed that polyphenols could significantly improve NAFLD. NAFLD was created by stimulating HepG2 cells with five mmol/L fructose for 48 h to develop the NAFLD model [39], and the results showed that l-carnitine could significantly reduce intracellular lipid levels and improve oxidative stress.
Due to their relative simplicity, single culture models have been widely used in studies, but they lack dimension, media flow, only one type of cells, lack of other types of cells, such as Kupffer cells, stellate cells, and macrophages, and lack of interactions between cells.
Co-culture Model
The co-culture model can make up for the defects of the single culture model. The second type of cells is introduced into the model, which increases the interaction between cells and can better simulate the physiological mechanism of the liver.
The co-culture of human primary hepatocytes and endothelial cells can support liver cells to maintain their phenotypic morphology, improve their specific functions, and form capillary vascular-like structures, which is more conducive to simulating the in vivo environment and studying the pathological mechanism of fatty liver disease [40]. Kupffer cell is a macrophage located in the hepatic sinusoid and can secrete inflammatory cytokines. The co-culture of human primary hepatocytes and Kupffer cells can assess hepatocyte response in a pro-inflammatory environment [41]. For 24 hours, they co-cultured human hepatocyte HuH7 and hepatic stellate cell LX2, which had been stimulated by fatty acids increased intracellular lipid accumulation, and α smooth muscle actin expression suggesting activation of hepatic stellate cells and the appearance of steatosis and fibrosis. In another study, human primary hepatocytes, hepatic stellate cells, and Kupffer cells were cultured together and stimulated with fatty acids, glucose, insulin, and inflammatory cytokines to simulate non-alcoholic fat hepatitis [42]. In this model, the de novo fat synthesis was enhanced. Oxidative stress, inflammation, fibrosis, and activation of hepatic stellate cells were observed in the cells [43]. HepG2 cells were co-cultured with THP-1 macrophages in a model developed by [44]. First, the macrophages were affixed to sterile slides, and then the slides were placed into 6-well plates inoculated with HepG2 cells, and the concentration of 1 mmol/L mixed fatty acids (PA and OA ratio is 1:2) were added. The NAFLD model was established 24 h after induction.
The advantages of the co-culture model are that it makes up for the deficiency of the single culture model, the culture conditions are simple, and high throughput experiments can be carried out. However, there are few kinds of co-culture models, and they have excellent development potential in the future.
The environment of the three-dimensional
The culture model is critical for cell survival. There is physical signal transmission between liver cells and extracellular matrix in living liver tissue, and the extracellular matrix also plays a role in supporting three-dimensional structure [45]. Human primary hepatocytes cultured for a long time in a traditional two-dimensional system undergo morphological alterations due to epithelial-mesenchymal transformation, resulting in hepatocyte polarity and related loss of liver function [46].
The commonly used methods of three-dimensional culture are scaffold culture and suspension culture. Hydrogel, collagen, and lamininare among the widespread used scaffold materials. Scaffolds can support liver cells and form similar interactions between cells and matrix. Suspension culture refers to the spontaneous aggregation of cells to form spheres under suspension conditions, which is simple to operate, cheap, and thus, widely used [47]. Three-dimensional culture is also divided into three-dimensional single cell culture and three-dimensional co-culture. The following is the description of the two.
(1) 3D single culture model
Even after 21 days of culture, the human primary hepatocyte aggregates enveloped in hydrogel scaffolds still maintained the same cell phenotype [48]. In another experiment, bioprinters simultaneously aggregated cellular aggregates with supporting structures (usual hydrogels) based on a specific liver-like design template [49]. Fatty acids were added to the model group, and insulin and glucose were administered to the control and model groups, respectively, to mimic the effects of insulin and glucose on human primary hepatocytes [50]. After seven days of incubation, lipid accumulation increased significantly in the model group after 14 days. The fatty acid consumption of the model group was more than four times that of the control group. There was no significant change in the leakage amount of AST and Alanine aminotransferase (ALT) in the cells after modeling, indicating that the activity of delicate cells was regular and not affected by the modeling solution. The modeling solution did not affect intracellular glutathione hydrolevel, LDH release, urea production, and mitochondrial activity, which proved that the cells in this three-dimensional model were fully functional. The expression levels of CYP2E1, IGFBI, PDK4, and CYP7A1 were all significantly increased, indicating that the model had the characteristics of steatosis. This model is effective in detecting dimethylformin and other anti-fatty liver drugs.
The three-dimensional model can better simulate the in vivo environment, and the culture time is longer, which is conducive to repeated induction experiments. The spherical 3D model can be used in high-throughput screening experiments with high efficiency. The downside is that building 3D models is time-consuming and expensive, and since it is still in its early stages of use, some of its features have yet to be proven.
(2) 3D co-culture model
Three-dimensional co-culture models can study more complex fatty liver phenotypes such as inflammation and liver fibrosis. In the three-dimensional co-culture model, human hepatocytes and parenchymal cells can be cultured together, simulating the interaction between various types of cells in the liver in a three-dimensional space. The advantage of the 3D co-culture model is that it can maintain important metabolic functions for a long time and induce the pathological manifestation of fatty liver under relevant conditions.
Primary human liver cells were co-cultured with stellate, Kupffer, and endothelial cells utilizing the 3D human liver microtissue system of the Swiss brand InSphero and effectively established a model of fatty liver [51]. Models showed that PA significantly increased the expression of inflammatory factors and pro-fibrosis genes (including collagen, α -smooth muscle actin, metalloproteinase-tissue inhibitor 1, platelet-derived growth factor receptor -β, and interleukin-8), resulting in liver microtissue damage. Activation of the transforming growth factor β pathway was also detected, and the model was further validated using GS-4997, an inhibitor of the apoptotic signal-regulated kinase ASK-1. Results showed that GS-4997 significantly reduced pa-induced inflammatory and fibrotic responses and decreased apoptosis and hepatic stellate cell activation.
The disadvantages of the 3D co-culture model are complex operation and high price, so it needs to continue to improve technology and function to be more widely used in scientific research. There are many cell types and different culture methods used in the model of fatty liver cells. Each cell model has its advantages and disadvantages; researchers can choose the appropriate cell model according to the experimental environment, research objectives, and other factors to study fatty liver.
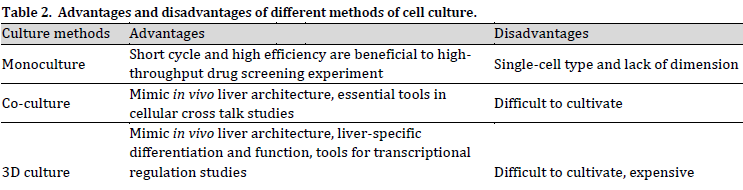
Intervention conditions of fatty liver cell model
Fatty liver induced by high fat
The medical fat emulsion has low toxicity, good solubility, easy operation, and economic benefit. NAFLD model was created utilizing HepG2 cells and a fat emulsion mass concentration of 0.2% to 4.0%. Cell activity was determined using the MTT method, intracellular lipids were stained with oil red O, and intracellular TG was determined using the kit method after 72 hours of incubation [52]. The results showed that 1% or lower fat emulsion did not affect cell viability, 0.4% or higher fat emulsion could gradually increase intracellular lipid droplets, and 1% was the best mass concentration when intracellular TG was the highest, and the survival rate of cells was the highest. PCR results showed that after incubation with 1% fat emulsion for 24 h, the expression levels of carnitine palmityl transferase one and TNF-α in the model group were significantly increased. In contrast, the expression levels of SrebP-1C were significantly decreased, suggesting the occurrence of NAFLD and inflammation.
PA and OA are the most abundant dietary long-chain fatty acids in the human body and are the most commonly used fatty acids in NAFLD modeling at present, but fatty acids exist in poor solubility. To better dissolve it in the medium, they used 5:1 ratios of BSA to a fatty acid to dilute it to the desired concentration after dissolving it in the medium [53]. Fatty liver was created by inducing WRL68 cells with 500 g/mL OA for 24 and 48 hours [54].[55] showed that the concentration ratio of PA to OA of 1:2 is the least toxic to hepatocytes, so many studies use this ratio. HepG2 cells were treated for 72 hours with OA and PA at concentrations of 0.2 mmol/L and 0.1 mmol/L, respectively, to create a model of fatty liver [56]. One mmol/L free fatty acid (OA: PA=2:1) was added to HepG2 and L02 cells for 24 hours to create fatty liver cell models [57].
Fatty liver induced by high glucose
Studies have proved that high fructose intake is associated with NAFLD and liver fibrosis [58]. They constructed a fructose-induced fatty liver model using L02 cells and found that fructose concentrations below 32 mmol/L had no influence on cell activity, and fructose concentrations not less than four mmol/L dramatically increased intracellular lipids [59]. The expression of acetyl-CoA carboxylase 1, SrebP-1, and carbohydrate reaction element-binding protein were up-regulated.
To study the modeling effects of fructose and fatty acids, they induced HepG2 cells for 24 h with 5-25 mmol/L glucose and 5-25 mmol/L fructose in the presence of 1 mmol/L mixed fatty acids (PA and OA concentration ratio of 1:2) [60]. Changes in lipid and uric acid production, glucose metabolism, oxidation status, and related genes and proteins were monitored. The results showed that PA-induced insulin resistance, oxidative stress, and lipid accumulation, OA promoted intracellular lipid accumulation. In contrast, fructose promoted the production of uric acid and cholesterol, and fructose, glucose, and fatty acid synergically increased intracellular TG and extracellular MDA.
Alcohol-induced fatty liver disease
The liver is the primary storage site of iron. When AFLD occurs in the body, iron content in the liver increases, and iron can promote lipid oxidation, thus damaging liver cells [61]. L02 cells were induced with a culture medium containing 0.5% ethanol and 0.01% ferrous sulfate, then changed to the culture medium without ethanol and ferrous sulfate for three days. After three days, repeated three times and obtained AFLD cell model. After ethanol and ferrous sulfate induction, intracellular TG content increased significantly, and lipid accumulation increased. However, AST andALT levels did not change significantly, indicating that a low alcohol concentration had no significant effect on cell activity [62].
When studying the effect of lactone on AFLD, they induced L02 cells with alcohol at a concentration of 100 mmol/L for 48 h and detected a significant increase in the contents of TG and TC in cells, resulting in the fatty liver [63]. HepG2 cells were co-cultured for 20 hours with curcumin (1mmol/L) in HepG2 cell media, which was then supplemented with anhydrous alcohol (0.6 percent) for four hours [64]. The leakage amounts of ALT, AST, and LDH were significantly increased in the model group, suggesting cell damage. TG, MDA, and alcohol dehydrogenase contents increased significantly, suggesting that lipid and lipid peroxidation products increased.
In summary, high fat, high-sugar, and alcohol can induce cell steatosis. Generally speaking, the higher the concentration, the longer the action time, the better the effect of inducing fatty liver, but high alcohol concentration will affect the survival rate of delicate cells. Different cell lines have different sensitivities to sugars, lipids, and alcohols, and the researchers need to pre-screen for the appropriate concentration of mold solution. The indicators of NAFLD include TG, TC, glycogen, ROS, and inflammatory factors. AFLD was detected by TG, TC, ROS, AST, ALT, MDA, alcohol dehydrogenase.
Conclusion
As described in this paper, cell models can reflect the pathogenesis of NAFLD and serve as a tool to test new treatments and prevention strategies. At present, there are many kinds of fatty liver cell models, among which the primary cell and immortal cell lines of two-dimensional culture are widely used. With the development of science and technology, cell models are becoming more complex and precise, such as three-dimensional culture and co-culture models, and liver biopsy technology has been improved. Fatty liver can be induced by high fat, sugar, and alcohol. Cell model has the advantages of a large number, short cycle, and easy to repeat, which is a powerful tool to study the pathogenesis of fatty liver and can complement animal model. The future goal should be to develop and improve the standardized cell model, explore the molecular mechanism of NAFLD development, and provide a better theoretical basis for treating fatty liver.
References
1 | Fan JG. Epidemiology of alcoholic and non-alcoholic fatty liver disease in China. J. Gastroenterol. Hepatol. 2013;28:11-7. DOI |
2 | Li J, Zou B, Yeo YH, Feng Y, Xie X, Lee DH, Fujii H, Wu Y, Kam LY, Ji F, Li X. Prevalence, incidence, and outcome of non-alcoholic fatty liver disease in Asia, 1999–2019: a systematic review and meta-analysis. Lancet Gastroenterol. Hepatol. 2019;4(5):389-98. DOI |
3 | Bibbò S, Ianiro G, Dore MP, Simonelli C, Newton EE, Cammarota G. Gut microbiota as a driver of inflammation in non-alcoholic fatty liver disease. Mediators Inflamm. 2018. DOI |
4 | Day CP, James OF. Steatohepatitis: a tale of two “hits”? Gastroenterology. 1998;114(4):842-5. DOI |
5 | Arab JP, Arrese M, Trauner M. Recent insights into the pathogenesis of non-alcoholic fatty liver disease. Annu. Rev. Pathol. 2018;13:321-50. DOI |
6 | Friedman SL, Neuschwander-Tetri BA, Rinella M, Sanyal AJ. Mechanisms of NAFLD development and therapeutic strategies. Nat. Med. 2018;24(7):908-22. DOI |
7 | Abdelmalek MF, Lazo M, Horska A, Bonekamp S, Lipkin EW, Balasubramanyam A, Bantle JP, Johnson RJ, Diehl AM, Clark JM, Fatty Liver Subgroup of the Look AHEAD Research Group. Higher dietary fructose is associated with impaired hepatic adenosine triphosphate homeostasis in obese individuals with type 2 diabetes. Hepatology. 2012;56(3):952-60. DOI |
8 | Ioannou GN. The role of cholesterol in the pathogenesis of NASH. Trends Endocrinol. Metab. 2016;27(2):84-95. DOI |
9 | Du Plessis J, Korf H, van Pelt J, Windmolders P, Vander Elst I, Verrijken A, Hubens G, Van Gaal L, Cassiman D, Nevens F, Francque S. Pro-inflammatory cytokines but not endotoxin-related parameters associate with disease severity in patients with NAFLD. PloS one. 2016;11(12):e0166048. DOI |
10 | Ogawa Y, Suganami T, Tanaka M, Itoh M. Parenchymal-stromal cell interaction in metabolic diseases. Inflamm. Regen. 2015;35(4):167-71. DOI |
11 | Silva AK, Peixoto CA. Role of peroxisome proliferator-activated receptors in non-alcoholic fatty liver disease inflammation. Cell. Mol. Life Sci. 2018;75(16):2951-61. DOI |
12 | Spencer MD, Hamp TJ, Reid RW, Fischer LM, Zeisel SH, Fodor AA. Association between composition of the human gastrointestinal microbiome and development of fatty liver with choline deficiency. Gastroenterology. 2011;140(3):976-86. DOI |
13 | Horie Y, Yamagishi Y, Ebinuma H, Hibi T. Obesity, type 2 diabetes, age, and female gender: significant risk factors in the development of alcoholic liver cirrhosis. Hepatol. Int. 2013;7(1):280-5. DOI |
14 | Lu FB, Hu ED, Xu LM, Chen L, Wu JL, Li H, Chen DZ, Chen YP. The relationship between obesity and the severity of non-alcoholic fatty liver disease: systematic review and meta-analysis. Expert Rev. Gastroenterol. Hepatol. 2018;12(5):491-502. DOI |
15 | Boyle M, Masson S, Anstee QM. The bidirectional impacts of alcohol consumption and the metabolic syndrome: cofactors for progressive fatty liver disease. J. Hepatol. 2018;68(2):251-67. DOI |
16 | Lebrun V, Molendi-Coste O, Lanthier N, Sempoux C, Cani PD, van Rooijen N, Stärkel P, Horsmans Y, Leclercq IA. Impact of PPAR-α induction on glucose homoeostasis in alcohol-fed mice. Clin. Sci. 2013;125(11):501-11. DOI |
17 | Altamirano J, Bataller R. Alcoholic liver disease: pathogenesis and new targets for therapy. Nat. Rev. Gastroenterol. Hepatol. 2011;8(9):491-501. DOI |
18 | Rasineni K, Thomes PG, Kubik JL, Harris EN, Kharbanda KK, Casey CA. Chronic alcohol exposure alters circulating insulin and ghrelin levels: role of ghrelin in hepatic steatosis. Am. J. Physiol. Gastrointest. Liver Physiol. 2019;316(4):G453-61. DOI |
19 | Liu J. Ethanol and liver: recent insights into the mechanisms of ethanol-induced fatty liver. World J. Gastroenterol. 2014;20(40):14672. DOI |
20 | Jia L, Chang X, Qian S, Liu C, Lord CC, Ahmed N, Lee CE, Lee S, Gautron L, Mitchell MC, Horton JD. Hepatocyte toll-like receptor 4 deficiency protects against alcohol-induced fatty liver disease. Mol. Metab. 2018;14:121-9. DOI |
21 | Seglen PO, Reith A. Ammonia inhibition of protein degradation in isolated rat hepatocytes: Quantitative ultrastructural alterations in the lysosomal system. Exp. Cell Res. 1976;100(2):276-80. DOI |
22 | Wanniger J, Neumeier M, Hellerbrand C. Lipid accumulation impairs adiponectin-mediated induction of activin A by increasing TGF beta in primary human hepatocytes. Biochim Biophys Acta Mol Cell Biol Lipids. 2011, 1811(10):626-33. DOI |
23 | Yuan H, Zhang X, Huang X, Lu Y, Tang W, Man Y, Wang S, Xi J, Li J. NADPH oxidase 2-derived reactive oxygen species mediate FFAs-induced dysfunction and apoptosis of β-cells via JNK, p38 MAPK and p53 pathways. PloS one. 2010;5(12):e15726. DOI |
24 | Zheng X, Zhao MG, Jiang CH, Sheng XP, Yang HM, Liu Y, Yao XM, Zhang J, Yin ZQ. Triterpenic acids-enriched fraction from Cyclocarya paliurus attenuates insulin resistance and hepatic steatosis via PI3K/Akt/GSK3β pathway. Phytomedicine. 2020;66:153130. DOI |
25 | Yin J, Luo Y, Deng H, Qin S, Tang W, Zeng L, Zhou B. Hugan Qingzhi medication ameliorates hepatic steatosis by activating AMPK and PPARα pathways in L02 cells and HepG2 cells. J. Ethnopharmacol. 2014;154(1):229-39. DOI |
26 | Liu Y, Wang D, Zhang D, Lv Y, Wei Y, Wu W, Zhou F, Tang M, Mao T, Li M, Ji B. Inhibitory effect of blueberry polyphenolic compounds on oleic acid-induced hepatic steatosis in vitro. J. Agric. Food Chem. 2011;59(22):12254-63. DOI |
27 | De la Monte SM, Longato L, Tong M, DeNucci S, Wands JR. The liver-brain axis of alcohol-mediated neurodegeneration: role of toxic lipids. Int. J. Environ. Res. Public Health. 2009:6(7):2055-75. DOI |
28 | Khamphaya T, Chukijrungroat N, Saengsirisuwan V, Mitchell‐Richards KA, Robert ME, Mennone A, Ananthanarayanan M, Nathanson MH, Weerachayaphorn J. Nonalcoholic fatty liver disease impairs expression of the type II inositol 1, 4, 5‐trisphosphate receptor. Hepatology. 2018;67(2):560-74. DOI |
29 | Michaut A, Le Guillou D, Moreau C, Bucher S, McGill MR, Martinais S, Gicquel T, Morel I, Robin MA, Jaeschke H, Fromenty B. A cellular model to study drug-induced liver injury in non-alcoholic fatty liver disease: application to acetaminophen. Toxicol. Appl. Pharmacol. 2016;292:40-55. DOI |
30 | Barbero-Becerra VJ, Giraudi PJ, Chavez-Tapia NC, Uribe M, Tiribelli C, Rosso N. The interplay between hepatic stellate cells and hepatocytes in an in vitro model of NASH. Toxicol. In Vitro. 2015;29(7):1753-8. DOI |
31 | Tsuruga Y, Kiyono T, Matsushita M, Takahashi T, Kasai H, Todo S. Establishment of immortalized human hepatocytes by introduction of HPV16 E6/E7 and hTERT as cell sources for liver cell-based therapy. Cell Transplant. 2008;17(9):1083-94. DOI |
32 | Mann DA. Human induced pluripotent stem cell-derived hepatocytes for toxicology testing. Expert Opin. Drug Metab. Toxicol. 2015;11(1):1-5. DOI |
33 | Graffmann N, Ring S, Kawala MA, Wruck W, Ncube A, Trompeter HI, Adjaye J. Modeling non-alcoholic fatty liver disease with human pluripotent stem cell-derived immature hepatocyte-like cells reveals activation of PLIN2 and confirms regulatory functions of peroxisome proliferator-activated receptor alpha. Stem Cells Dev. 2016;25(15):1119-33. DOI |
34 | Parafati M, Kirby RJ, Khorasanizadeh S, Rastinejad F, Malany S. A non-alcoholic fatty liver disease model in human induced pluripotent stem cell-derived hepatocytes, created by endoplasmic reticulum stress-induced steatosis. Dis. Model. Mech. 2018;11(9). DOI |
35 | Alzebdeh DA. Development of scaffold architectures and heterotypic cell systems for hepatocyte transplantation. Wayne State University. 2015. |
36 | Schaffert CS, Duryee MJ, Bennett RG, DeVeney AL, Tuma DJ, Olinga P, Easterling KC, Thiele GM, Klassen LW. Exposure of precision-cut rat liver slices to ethanol accelerates fibrogenesis. Am. J. Physiol. Gastrointest. Liver Physiol. 2010;299(3):G661-8. DOI |
37 | Prins GH, Luangmonkong T, Oosterhuis D, Mutsaers HA, Dekker FJ, Olinga P. A pathophysiological model of non-alcoholic fatty liver disease using precision-cut liver slices. Nutrients. 2019;11(3):507. DOI |
38 | Rafiei H, Omidian K, Bandy B. Dietary polyphenols protect against oleic acid-induced steatosis in an in vitro model of NAFLD by modulating lipid metabolism and improving mitochondrial function. Nutrients. 2019;11(3):541. DOI |
39 | Montesano A, Senesi P, Vacante F, Mollica G, Benedini S, Mariotti M, Luzi L, Terruzzi I. L-Carnitine counteracts in vitro fructose-induced hepatic steatosis through targeting oxidative stress markers. J. Endocrinol. Invest. 2020;43(4):493-503. DOI |
40 | Salerno S, Campana C, Morelli S, Drioli E, De Bartolo L. Human hepatocytes and endothelial cells in organotypic membrane systems. Biomaterials. 2011;32(34):8848-59. DOI |
41 | Nguyen TV, Ukairo O, Khetani SR, McVay M, Kanchagar C, Seghezzi W, Ayanoglu G, Irrechukwu O, Evers R. Establishment of a hepatocyte-kupffer cell co-culture model for assessment of pro-inflammatory cytokine effects on metabolizing enzymes and drug transporters. Drug Metab. Dispos. 2015;43(5):774-85. DOI |
42 | Giraudi PJ, Becerra VJ, Marin V, Chavez-Tapia NC, Tiribelli C, Rosso N. The importance of the interaction between hepatocyte and hepatic stellate cells in fibrogenesis induced by fatty accumulation. Exp. Mol. Pathol. 2015;98(1):85-92. DOI |
43 | Feaver RE, Cole BK, Lawson MJ, Hoang SA, Marukian S, Blackman BR, Figler RA, Sanyal AJ, Wamhoff BR, Dash A. Development of an in vitro human liver system for interrogating non-alcoholic steatohepatitis. JCI insight. 2016;1(20). DOI |
44 | Chen Y, Ma K. NLRC4 inflammasome activation regulated by TNF-α promotes inflammatory responses in non-alcoholic fatty liver disease. Biochem. Biophys. Res. Commun. 2019;511(3):524-30. DOI |
45 | Ebrahimkhani MR, Neiman JA, Raredon MS, Hughes DJ, Griffith LG. Bioreactor technologies to support liver function in vitro. Adv. Drug Deliv. Rev. 2014;69:132-57. DOI |
46 | Kamei KI, Yoshioka M, Terada S, Tokunaga Y, Chen Y. Three-dimensional cultured Liver-on-a-Chip with mature hepatocyte-like cells derived from human pluripotent stem cells. Biomed. Microdevices. 2019;21(3):1-9. DOI |
47 | Bokhari M, Carnachan RJ, Cameron NR, Przyborski SA. Culture of HepG2 liver cells on three dimensional polystyrene scaffolds enhances cell structure and function during toxicological challenge. J. Anat. 2007;211(4):567-76. DOI |
48 | Gevaert E, Dolle L, Billiet T, Dubruel P, van Grunsven L, van Apeldoorn A, Cornelissen R. High throughput micro-well generation of hepatocyte micro-aggregates for tissue engineering. PloS one. 2014;9(8):e105171. DOI |
49 | Bhise NS, Manoharan V, Massa S, Tamayol A, Ghaderi M, Miscuglio M, Lang Q, Zhang YS, Shin SR, Calzone G, Annabi N. A liver-on-a-chip platform with bioprinted hepatic spheroids. Biofabrication. 2016;8(1):014101. DOI |
50 | Kostrzewski T, Cornforth T, Snow SA, Ouro-Gnao L, Rowe C, Large EM, Hughes DJ. Three-dimensional perfused human in vitro model of non-alcoholic fatty liver disease. World J. Gastroenterol. 2017;23(2):204. DOI |
51 | Mukherjee S, Zhelnin L, Sanfiz A, Pan J, Li Z, Yarde M, McCarty J, Jarai G. Development and validation of an in vitro 3D model of NASH with severe fibrotic phenotype. Am. J. Transl. Res. 2019;11(3):1531. |
52 | Vlaardingerbroek H, Ng K, Stoll B, Benight N, Chacko S, Kluijtmans LJ, Kulik W, Squires EJ, Olutoye O, Schady D, Finegold ML. New generation lipid emulsions prevent PNALD in chronic parenterally fed preterm pigs. J. Lipid Res. 2014;55(3):466-77. DOI |
53 | Fujimoto Y, Onoduka J, Homma KJ, Yamaguchi S, Mori M, Higashi Y, Makita M, Kinoshita T, Noda JI, Itabe H, Takanoa T. Long-chain fatty acids induce lipid droplet formation in a cultured human hepatocyte in a manner dependent of Acyl-CoA synthetase. Biol. Pharm. Bull. 2006;29(11):2174-80. DOI |
54 | Chen KY, Lin JA, Yao HY, Hsu AC, Tai YT, Chen JT, Hsieh MC, Shen TL, Hsu RY, Wu HT, Wang GH. Arctigenin protects against steatosis in WRL68 hepatocytes through activation of phosphoinositide 3-kinase/protein kinase B and AMP-activated protein kinase pathways. Nutr. Res. 2018;52:87-97. DOI |
55 | Gomez-Lechon MJ, Donato MT, Martínez-Romero A, Jiménez N, Castell JV, O’Connor JE. A human hepatocellular in vitro model to investigate steatosis. Chem. Biol. Interact. 2007 Jan 30;165(2):106-16. DOI |
56 | Qiu PL, Liu SY, Bradshaw M, Rooney-Latham S, Takamatsu S, Bulgakov TS, Tang SR, Feng J, Jin DN, Aroge T, Li Y. Multi-locus phylogeny and taxonomy of an unresolved, heterogeneous species complex within the genus Golovinomyces (Ascomycota, Erysiphales), including G. ambrosiae, G. circumfusus and G. spadiceus. BMC Microbiol. 2020;20(1):1-6. DOI |
57 | Yang Y, Zhang W, Wu X, Wu J, Sun C, Luo F, Pei Z. Systemic Overexpression of GDF5 in Adipocytes but Not Hepatocytes Alleviates High-Fat Diet-Induced Nonalcoholic Fatty Liver in Mice. Can. J. Gastroenterol. Hepatol. 2021;2021. DOI |
58 | Abdelmalek MF, Suzuki A, Guy C, Unalp‐Arida A, Colvin R, Johnson RJ, Diehl AM, Nonalcoholic Steatohepatitis Clinical Research Network. Increased fructose consumption is associated with fibrosis severity in patients with non-alcoholic fatty liver disease. Hepatology. 2010;51(6):1961-71. DOI |
59 | Huang XJ, He CJ, Liang S, Wang J, Li J, Yang GZ, Zhao Z. Veratrilla baillonii Franch Could Alleviate Lipid Accumulation in LO2 Cells by Regulating Oxidative, Inflammatory, and Lipid Metabolic Signaling Pathways. Front. Pharmacol. 2020;11:1511. DOI |
60 | Zhao L, Guo X, Wang O, Zhang H, Wang Y, Zhou F, Liu J, Ji B. Fructose and glucose combined with free fatty acids induce metabolic disorders in HepG2 cell: A new model to study the impacts of high‐fructose/sucrose and high‐fat diets in vitro. Mol. Nutr. Food Res. 2016;60(4):909-21. DOI |
61 | Djuric Z, Potter DW, Taffe BG, Strasburg GM. Comparison of iron‐catalyzed DNA and lipid oxidation. J. Biochem. Mol. Toxicol. 2001;15(2):114-9. DOI |
62 | Xue Y, Wang J, Huang Y, Gao X, Kong L, Zhang T, Tang M. Comparative cytotoxicity and apoptotic pathways induced by nanosilver in human liver HepG2 and L02 cells. Hum. Exp. Toxicol. 2018;37(12):1293-309. DOI |
63 | Jin X, Chen K, Lv ZY, Zheng L, Liu YP, Chen SH, Yu CH, Jiang XY, Zhang CY, Li YM. HDMCP uncouples yeast mitochondrial respiration and alleviates steatosis in L02 and hepG2 cells by decreasing ATP and H2O2 levels: a novel mechanism for NAFLD. J. Hepatol. 2009;50(5):1019-28. DOI |
64 | Moscato S, Ronca F, Campani D, Danti S. Poly (vinyl alcohol)/gelatin hydrogels cultured with HepG2 cells as a 3D model of hepatocellular carcinoma: a morphological study. J. Funct. Biomater. 2015;6(1):16-32. DOI |
Cite this article:
Khan, S., Khan, M. Molecular developments in cell models of fatty liver disease. DYSONA – Life Science, 2022;3(1): 16-29. doi: 10.30493/dls.2022.325915