Francesco Zagami 1,2*
1, Tabaka Mission Hospital, Tabaka, Kisii, Kenia
2, Independent Researcher and Diagnostic Consultant, Medicitalia Srl, Foro Bonaparte, Milano, Italy
E-mail:
francesco_zagami@virgilio.it
Received: 03/12/2022
Acceptance: 28/12/2022
Available Online: 30/12/2022
Published: 01/04/2023
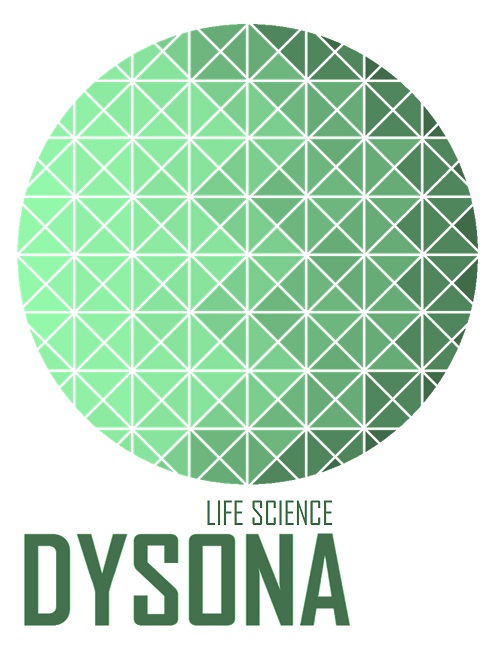
Manuscript link
http://dx.doi.org/10.30493/DLS.2022.301222
Abstract
Sarcopenia and frailty are two geriatric syndromes that cause loss of muscle mass and function in addition to other various impairments. Sarcopenia is a specific phenotype of frailty where low grip strength, slow walking, and reduced muscle mass are observed. Since modern communities are aging more than ever, geriatric syndromes have become more prevalent and are linked to morbidity and disabilities. Several mechanisms are involved in the pathophysiology of sarcopenia, such as autophagy, protein synthesis and degradation, satellite cell activation damages, mitochondrial dysfunction, increased intracellular oxidative stress, and other factors like malnutrition, physical inactivity, and systemic inflammation that contribute to muscle weakness and degeneration. Modulating factors such as exosomes and transporters that can govern muscle loss and regeneration are involved in this pathogenetic pathway. The significance of these compounds is not theoretical since they provide a useful diagnostic tool as well as a potential treatment target. Thereby, the study of exosomes can explain the complex and intricate process of geriatric syndrome as an increasingly frequent complication of various diseases in the elderly. Therefore, this study explores the overlapping pathophysiological pathways between chronic diseases and sarcopenia by reviewing the current conception of exosome production and exosomal cargo in chronic diseases.
Keywords: Geriatric syndrome, Dementia, Pancreatic cancer, Stroke, Pathophysiological pathway, Exosomes
Introduction
Approximately 25% of the population will be older than 60 in 2050, and this demographic transition will lead to an increase in age-related diseases [1][2]. Besides the major chronic conditions (CCs) common in the elderly, such as cardiovascular disease, diabetes, strokes, cancer, chronic obstructive airway disease, musculoskeletal conditions, and dementia, geriatric syndromes (GS) should be considered since they accompany many of these medical conditions [3].
Frailty and sarcopenia are included in the category of GS. While frailty is defined as a considerable reduction in the performance of numerous organ systems, resulting in the individual’s excessive sensitivity to endogenous and external stresses, sarcopenia is a subset of frailty characterized by progressive and extensive skeletal muscle impairments. Both situations increase the likelihood of faster functional decline and negative health consequences [4].
The incidence of GS can occur before or simultaneously with the clinical onset of the CC. However, there is the possibility that GSs can manifest themselves in the pathogenetic pathways of CCs as a related factor, especially in systemic diseases such as neurological, neoplastic, organ failure, arteriosclerosis, and diabetes [5]. Therefore, their presence determines a worsening of the health-related quality of life and clinical management of old patients [6]. Considering GSs as an inducing/worsening factor, their appearance can be linked to a pathophysiological mechanism shared with CCs.
In relation to the more prevalent CCs in the elderly, it was claimed that eliminating exposure to 12 recognized risk factors might postpone or even prevent up to 40% of CCs occurrences. Smoking, hearing issues, elevated blood pressure, diabetes, overweight, depression, lack of physical activity, social isolation, heavy drinking, brain injury, and air pollution are among these risk factors [7]. Thus, physical inactivity represents a fil rouge between CCs and GSs and can facilitate the development or worsen of the pathological state.
Various factors are considered when evaluating for frailty phenotype construct. These factors are weakness (assessed by the strength of the handgrip), sluggishness (assessed by movement speed), reduced physical activity, unintentional weight loss, and self-reported weariness [8][9]. The overlap between frailty and sarcopenia creates further uncertainties when analyzing patients who also manifest cachexia or malnutrition since all these cases appear to occur concurrently [10]. This overlapping complicates the understanding of the physiopathological pathway between GSs and CCs when simultaneously present. In particular, when there is a high overlap of diagnosis, it is necessary to focus on the patients, thus allowing to catch nuances that are more objective than subjective evaluations.
Recent statistics show increasing overlaps between GSs and CCs [11]. Furthermore, these numbers are expected to increase dramatically in the following 20-30 years [12]. Therefore, this article aims to analyze the current conception of physiopathological pathways between CCs and the appearance of sarcopenia.
Dementia and sarcopenia
The cognitive frailty (CF) was operationally defined when mild cognitive impairment (MCI) and physical frailty occur in the absence of dementia [13]. Despite having a possibly reversible loss in cognitive reserve, people with CF have a greater chance of acquiring dementia [14]. This places CF in between age-related cognitive impairments and neurodegenerative disorders [15].
An increased risk of frailty-malnutrition is observed in depressed patients as a consequence of dementia, and a high prevalence of sarcopenia can also be observed as the disease progresses [16]. Furthermore, an accelerated impairment of cognitive changes (a decline in memory, logic, social interaction, decision-making, and language-related abilities) is observed in the sarcopenia progression [17].
In dementia physiopathogenesis, neuroinflammation in response to pathogens or a number of pathophysiological processes (including excitotoxicity, mitochondrial dysfunction, and oxidative stress) results in stress or damage to neural, glial, and vascular endothelial cells. These stresses and injuries constitute the first occurrence of vascular dementia [18][19]. The cascade involvement of all these components leads to neuronal death, followed by the release of mitochondrial DNA (mtDNA) from the cells by extracellular vesicles (EVs). The circulating cell-free mtDNA molecules serve as damage-related molecular patterns (DAMPs), representing a functional connection between systemic inflammation and mitochondrial damage [20] (Fig. 1). This release can also occur when mitochondrial depolarization is insufficient. Cells may either postpone autophagy to remove slightly damaged organelles or switch from mitophagy to mitochondrial component extrusion within EVs [21]. The involvement of chronic cerebral hypoperfusion in the medial prefrontal area is associated with nutritional apathy [22] and represents a pathophysiological factor that decreases food intake, evolving into nutritional deficiency, which is worsened by the increase in anorexic cytokines [23][24].
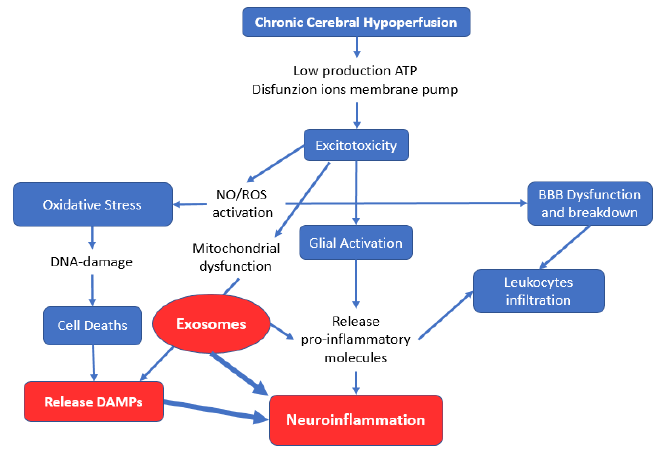
Microglia activated by excitotoxicity release exosomes carrying the proinflammatory marker miR-21, which can be considered an exosomal biomarker of microglia activation [25]. Furthermore, proinflammatory stimuli (lipopolysaccharides, interleukin 4, and interleukin 10) result in exosome release increments and changes in exosome content [26][27][28]. The exosomes produced by neurons in major depressive disorder (MDD) were recently found to contain miR‑9‑5p, which polarizes microglia in M1 cells to induce inflammation [29]. This inflammatory inductor determines systemic inflammation that underlies sarcopenia of muscle loss in normal aging, where atrophy is caused by ubiquitin-proteasome system activation and NF–κB signaling [30]. This systemic inflammation plays an essential role in the development of sarcopenia. The chronic low-grade inflammation in dementia combined with the body composition changes linked to malnutrition can be interconnected phenomena that characterize the aging-disease process, leading to sarcopenia [31].
Sarcopenia was found to have a positive correlate with levels of Interleukin 6 (IL-6), Interleukin 10 (IL-10), and IL-6/IL-10 ratios, but a negative correlation with body mass index (BMI) [32][33]. The value of BMI in sarcopenic obesity, on the other hand, is negatively influenced by the development of proinflammatory cytokines by macrophage (IL-6) and C-RP by the liver. As a result, muscle mass loss is substantially associated with increasing fat mass rather than changes in BMI. Individuals with CF or moderate to severe depression were found to have higher levels of IL-6, which has been shown to have a deleterious influence on central dopaminergic function, resulting in weariness, motor skill difficulty, and cognitive decline [13][34].
The documented presence of a muscle-brain endocrine pathway controlled by myokines helps to explain how muscular deterioration can alter cognitive states [35]. These muscle-produced substances enhance memory and protect against neural damage [36]. Sarcopenia is also associated with a decrease in the regenerative ability of skeletal muscle stem cells, as well as an altered pace of tissue regeneration and differentiation, which may result in impaired myokine synthesis and secretion, with a deleterious impact on brain function [37]. Tumor necrosis factor-α (TNF-α), IL-10, and Interleukin-1 (IL-1) are inhibited by myokines generated during muscular contraction (Fig. 2). Irisin, myostatin, and Interleukin-15 work together to regulate muscle-adipose tissue communication [38]. During a contraction, muscle also secretes fibroblast growth factor 21 (FGF-21), Interleukin-8, leukaemia inhibitory factor (LIF), angiopoietin-like 4 (ANGPTL4), follistatin-like 1 (FSTL1), vascular endothelial growth factor (VEGF), and brain-derived neurotrophic factor (BDNF) [39]. High circulating BDNF levels have been linked to a lower incidence of dementia [40].
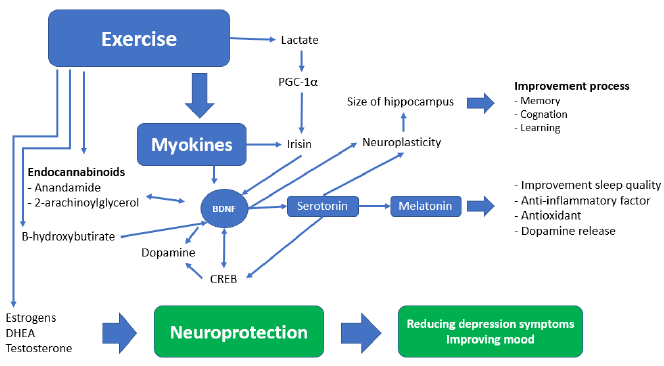
The molecular mechanisms that connect the loss of muscle mass with cognitive decline include a systemic inflammation determined through altered quality/quantity of brain myokine secretion by activated microglia, insulin resistance, anabolic resistance, oxidative stress, and mitochondrial dysfunction. In this pathway, nutrition should not be ignored since it is a vital element of the induction and recovery mechanism through the exosomes of food [41]. The production and secretion of myokines in grafted sarcopenia have negative consequences on the brain function, which can be called “neurogenic syndrome” [36][42].This increased myokine production intensifies inflammation and worsens muscle glucose metabolism, potentially influencing insulin transportation throughout the blood-brain barrier and decreasing the prevalence of insulin receptors in the brain as we age. Poor mobility and decreased function of the lower limbs may occur years before the onset of dementia, resulting in a swift evolution towards prolonged bed rest which accelerates the sarcopenic process.
In dementia, cerebral hypoperfusion, accompanied by the consequent increase in free radicals and mitochondrial dysfunction, represent the primum movens of the chain of events leading to sarcopenia. The locosystemic progression of the disease is linked to the exosomes that perform a pivotal pathophysiological role in the development of neurodegenerative disorders and involve in skeletal muscle regeneration. Nevertheless, exosomes have shown the potential to carry therapeutic cargo, with promising future therapeutic and diagnostics applications in neurodegenerative disorders prior to the emergence of symptoms.
Cancer and sarcopenia
Cancer is largely related to older age since newly diagnosed cases, and deaths are usually observed in individuals over the age of 65 [44], and it is anticipated that by 2030, adults over the age of 65 will account for 70% of all newly diagnosed cases [45]. Similarly, sarcopenia in oncology is common in older persons and has been linked to chemotherapeutic toxicity and surgical complications [46]. Neoplasms with high sarcopenia prevalence (>50%) are Urothelial, oesophageal, colon, prostate, and thyroid cancers. Whereas the prevalence of sarcopenia varies between 35% and 50% in head and neck squamous cell carcinoma in addition to lung, pancreatic, and ovarian cancers. Lower prevalence (<35%) are observed in breast, gastric, colorectal, and hepatocellular cancers [47]. However, these outcomes still vary based on disease stage and even diagnosis method. For instance, 30-65 % of the patients with pancreatic cancer showed symptoms of sarcopenia [48]. The same study reported that when it is present, sarcopenia results in more severe outcomes such as physical disability, lengthy hospital stays, infections, poor tolerance to chemotherapy, and reduced survival [48].
Cancer-associated sarcopenia can be considered a complex metabolic syndrome in which distinctive pathophysiological elements are associated with the degradation of cellular components, producing an exceptional inflammatory secretome and a strong mitochondrial dysfunction [49]. In the early phase of pancreatic ductal adenocarcinoma (PDAC), the increased level of circulating branched-chain amino acids (BCAAs; isoleucine, leucine, and valine) and monocyte chemoattractant protein-1 (MCP-1) are early warnings of sarcopenia [50][51]. Cancer and the host immune system generate several inflammatory cytokines, including IL-1, IL-6, IL-8, and TNF-α. TNF-α promotes lipid metabolism, protein denaturation, insulin resistance, and muscular atrophy. When the effect of TNF-α is combined with IL-1, IL-6, and the oxidative stress-dependent product nuclear factor-kB (NF-kB), the ubiquitin-proteasome pathway is activated, resulting in the degradation of regulatory proteins [52]. When activated, NF-kB suppresses the production of myoblast determinant protein 1 (MyoD), a myoprotein involved in cell proliferation following muscle injury, hence impairing myocytes’ capacity to repair themselves [53]. TNF-α is also linked to the IGF-1/PI3K/Akt signaling inhibition, which reduces muscle anabolic ability [54]. Increased IL-6 levels are associated with sarcopenia, extreme tiredness, and rapid weight loss. Additionally, IL-6 cooperates with TNF-α to stimulate the Janus kinase (JAK)-signal transducer and activator of transcription (STAT) pathway, which is implicated in inflammation, cancer development, and muscle mass atrophy [54]. Moreover, TNF-α and IL-6 both block myocyte development, resulting in ultrastructural myofiber degradation and the replacement of muscle mass with collagen and adipose tissue [54]. This pathway is also maintained by free fatty acids generated by lipolysis that stimulate the release of the ubiquitin ligases Atrogin-1 and MuRF1 [55].
The proinflammatory cytokines in pancreatic cancer, particularly IL-1, may also have a direct impact on the pathways underlying the central anorexia process by increasing the production of hypothalamic serotonin, which is necessary for the activation of proopiomelanocortin (POMC)/CART (cocaine- and amphetamine-regulated transcript) neurons [56]. Numerous miRNAs found in tumor-derived exosomes have been shown to exert effects on muscle cells and cause cancer-related muscle wasting by promoting inflammatory production, activating catabolism, and even regulating cellular breakdown pathways [57].
Exosomes are a class of extracellular vehicles (EVs) that are defined as membrane-bound nanovesicles of endocytic origin, with a diameter of 40–150 nm. These structures are composed of nucleic acids [DNA, mRNA, miRNA, and long and short non-coding RNAs], proteins [cytoskeletal proteins, transmembrane proteins, and heat shock proteins (HSPs)], and enzymes [GAPDH, ATPase, phosphoglycerate kinase 1 (pgk1), and RAB] [58]. For example, pancreatic cancer cells create exosomes that contain specifically miR-21. The production of miR-21 is increased by the production of TGF-β by M2 macrophage that invades the tumor inducing the death of muscle cells. This phenomenon takes place as a result of miR-21 ability to bind to and activate toll-like receptors promoting cell death through the activation of the c-Jun N-terminal kinase pathway (JNK) [57]. Recently, it was discovered that a zinc transporter known as ZIP4, which acts as a regulator of the growth of pancreatic tumours, plays a key role in the cachexia that is associated with pancreatic cancer. This transporter promotes the release of heat shock proteins 70 and 90 via EV activating toll-like receptor 4 (TLR4) and stimulating mitogen-activated protein kinase 14 (p38 MAPK)-mediated muscle breakdown [59]. According to the findings of the same study, knocking down ZIP4 boosted survival while reducing the loss of body weight and muscle mass. In fact, exosomes generated from the PDAC are capable of suppressing glucose intake and lipidosis (Fig. 3). Additionally, these exosomes have the ability to stimulate the movement of glucose transporter 4 protein (Glut4) from the cell surface to the plasma membrane. This movement facilitates the diffusion of circulating glucose across the concentration gradient into muscle and fat tissues [60].
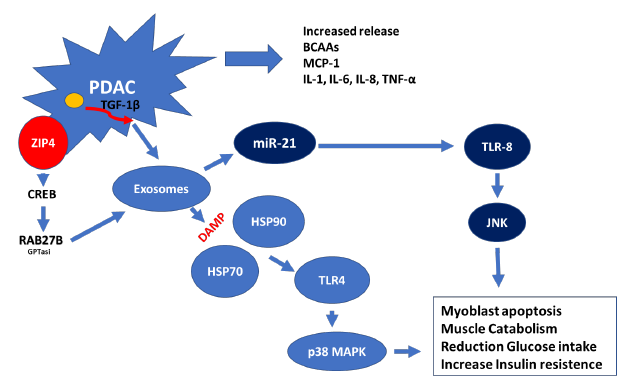
It is possible to draw the conclusion that PDAC produced exomes and their content operate as the most important facilitator of intercellular communication. The fact that these molecules can target, control, program, and re-program their immediate microenvironment of organs and skeletal muscles provides a deeper understanding of the connection between cancer and sarcopenia.
Stroke and sarcopenia
Stroke is one of the world’s leading causes of death and disability [61]. It is forecasted that stroke cases will triplicate in 2050 compared to 2010, with 1/3 of total cases in the >85 age group and almost 2/3 of stroke cases in those older than 75 [62]. Furthermore, stroke incidence in older people correlates with higher mortality and poorer outcome [63]. In fact, shortly after the onset of and up to 28 days after an ischemic stroke (IS) episode, neurons and glial cells in the involved area produce DAMPs activating astrocytes that secrete proinflammatory cytokines (IL-1β), chemokines, metalloproteinases and matrix metalloproteinases (MMPs) that contribute to the damage of the BBB [64]. If astrocytes polarize, they can uptake extracellular glutamate, changing their phenotype and secreting neurotrophic factors that protect the damaged brain tissue [65]. Likewise, quiescent microglial cells are activated a few minutes after IS onset through exosome release, which, when containing miR-21-5p, promotes polarization in M1 phenotypes. The latter secrete IL-1β, IL-6, MMP-9, and TNF-α/ROS that induce inflammation and neurotoxic substances that aggravate brain damage, leakage of BBB, and neuronal apoptosis [66]. On the other hand, In the presence of an exosome transporting, miR-124-3p polarizes M2 microglia, which is an anti-inflammatory phenotype that secretes anti-inflammatory
cytokines (IL-4, IL-10, TGF-β) and activates T regulatory cells (CD4+ CD25+), which modulate immune reactions and reduce the inflammatory response, facilitating brain function recovery and improving the prognosis of stroke [67]. This polarization is oriented with the presence of several factors (IL-1, IL-6, IL-13, IFN-γ, IL-15, TXA2, and C1q) that promote microglia in M1 polarization or M2 microglia conversion to M1, while IL-4, IL-13, TGF-β, P2X4, and Nrf2 promote the conversion of both M2 and M1 microglia (Fig. 4 and Fig. 5) [67].
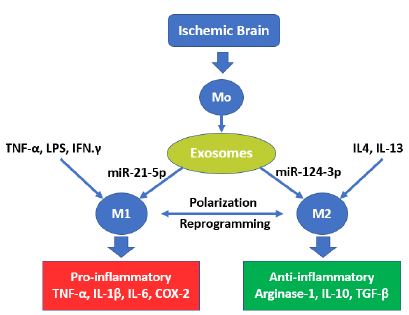
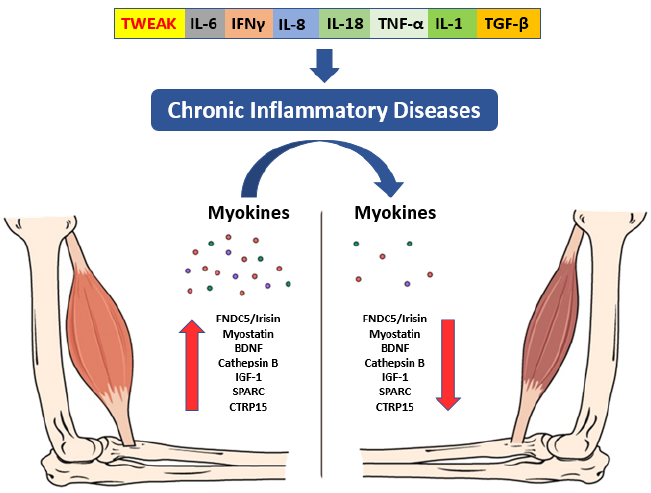
During the progression of cerebral ischemic stroke, there is an increase in the production of growth and trophic factors in addition to chemokines from the ischemic area [68]. These factors include the vascular endothelial growth factor (VEGF), fibroblast growth factor-2 (FGF-2), insulin-like growth factor-1 (IGF-1), brain-derived neurotrophic factor (BDNF), monocyte chemoattractant protein-1 (MCP-1), stromal cell-derived factor 1 (SDF1), endothelial nitric oxide synthase (eNOS), and angiopoietin-1/Tie2 (Ang1/Tie2) [68]. Some of these factors have many functions and encourage the development of new neurons, blood vessels, and oligodendrocytes [68]. The decreased trophic and regenerative EXOs and the motoneuronal damage that occurs during stroke negatively influence brain-muscle communication leading to the muscle becoming flaccid [69]. In fact, aging results in a natural gradual decline in communication between the central nervous system and skeletal muscle. This failure in communication leads to slow movement, weakness, increased falling tendency, and eventually a decline in function, a condition worsened by the ischemic event. This communication is bidirectional between the brain and skeletal muscle, and an important role is played by EVs, where the EXOs represent the essential components [70].
Upregulation of proinflammatory cytokine production that cross the blood brain barrier (BBB) leads the imbalanced secretion in the skeletal muscle of myokines and to muscle memory impairment. Lowering of the muscle myokine levels in blood, induced by sarcopenia, leads to reduce brain function through paracrine and autocrine manners. Failure of the brain-muscle axis is a direct result of ageing and this produces the major motor impairments which frequently accompany it. Many cytokines exhibit the pleiotropy (exert many different types of responses, often on different cell types) and redundancy (different cytokines can induce similar signals) phenomena, in which the circulating levels of cytokines make the difference. Cytokines not only can have overlapping actions but can also share receptor components (cytokine receptor pleiotropy and cytokine receptor redundancy). For example, modest levels of IL-6 may stimulate satellite cell activation and myotube regeneration, whereas chronically increased levels of IL-6 synthesis induce muscle tissue atrophy. These different effects may be explained by a cross-talk between the IL-6/IL-6 receptor and gp130 trans-signalling pathways, which counter the traditional IL-6 receptor signalling pathway’s regenerative and anti-inflammatory properties. The exosomes released from the ischemic areas contain numerous molecules, including microRNAs (miRs), which can regulate the expression of genes involved in the fine regulation of numerous physiological processes, including muscle regeneration [71]. A number of studies have pointed to the significance of microRNAs, or miRs, in skeletal muscle functions and disorders. This is due to the fact that abnormal expression of miRs has been linked to a number of conditions affecting the skeletal muscle, including sarcopenia [72]. Expression of miRNAs such as miR-497, miRNA 21, and miR-99a in IS attenuates ischemic volumes and It prevents apoptosis in neuronal cells, which is essential for maintaining neurological processes, whereas overexpression of miR-let-7c-5p and miR-424 reduces the activation of microglia [73]. In this case, the extent of damage is correlated with the level of miRNAs expressed in the blood after IS and crossing BBB [74]. Up-regulation of miR-103, miR-103, miR-132, and miR-126 in intracerebral hemorrhage diminishes neurobehavioral and neuropathological alterations, which protects the integrity, attenuates neuroinflammation, and decreases neuronal apoptosis [75].
It is evident the exosomes released upon ischemia can play a vital role in post-stroke injury prevention or induction and development of geriatric syndromes such as sarcopenia. Therefore, these molecules can be considered valuable diagnostic and therapeutic tools in post-stroke rehabilitation.
Conclusion and future aspects
The malfunctioning of mitochondria and inflammation throughout the body are important contributors to the development of sarcopenia. On the other hand, our knowledge of the molecular factors that link the two systems is limited. Exosome-derived molecules have been shown to include cell-free mitochondrial DNA (mtDNA), which has been identified as one of the components that may behave as damage-associated molecular patterns (DAMPs).Among these molecules, non-coding miRNA represents the critical reunion point between mitochondrial dysfunction and chronic inflammation, with different types resulting in different outcomes. The importance of these molecules in the pathogenesis of chronic diseases discussed here represents only the tip of the iceberg of their participation in many, if not all, acute and chronic diseases. Thus, exosomes have acquired the pathophysiological audience nowadays as reliable biomarkers. The high heterogeneity of exosomes reflects the pathophysiological conditions of the cells from which they originate. Then, their cargo is a potential organ biomarker for diagnosis and clinical evaluation in the diagnostics of various diseases. For instance, a 100% accuracy in the diagnosis of PDAC was obtained using the CA19-9 marker in combination with miRNAs-21, -210 [76][77].
Furthermore, targeting the exosomal secretions is now considered for its therapeutic application not only to stop the evolution of sarcopenia [78] but also to treat various diseases, including those considered here. Integrating engineered exosomes as “Trojan Horses” can be applied in various human chronic and infectious diseases [79], rendering it a promising therapeutic method in the near future.
Conflict of interest statement
The author declared no conflict of interest.
Funding statement
The author declared that no funding was received in relation to this manuscript.
Data availability statement
The author declared that no data was used in the preparation of this article.
References
1 | Kanasi E, Ayilavarapu S, Jones J. The aging population: demographics and the biology of aging Periodontol. 2000. 2016;2(1):13-8. DOI |
2 | Maklakov AA, Chapman T. Evolution of ageing as a tangle of trade-offs: energy versus function. Proc. R. Soc. 2019. DOI |
3 | Inouye SK, Studenski S, Tinetti ME, and Kuchel GA. Geriatric Syndromes: Clinical, Research and Policy Implications of a Core Geriatric Concept. J. Am. Geriatr. Soc. 2007;55(5):780–91. DOI |
4 | Anpalahan M, Gibson SJ. Geriatric syndromes as predictors of adverse outcomes of hospitalisation. Inter. Med. J. 2008;38(1):16-23. DOI |
5 | Harrison C, Fortin M, van den Akker M, Mair F, Calderon-Larranaga A, Boland F, Wallace E, Jani B, and Smith S. Comorbidity versus multimorbidity: Why it matters. J. Comorb. 2021;11. DOI |
6 | Bulut EA, Soysal P, and Isik AT. Frequency and coincidence of geriatric syndromes according to age groups: single-center experience in Turkey between 2013 and 2017. Clin. Interv. Aging. 2018;13:1899–905. DOI |
7 | Livingston G, Huntley J, Sommerlad A, Ames D, Ballard C, Banerjee S, Brayne C, Burns A, Cohen-Mansfield J, Cooper C, Costafreda SG, Dias A, Fox N, Gitlin LN, Howard R, Kales HC, Kivimäki M, Larson EB, Ogunniyi A, Orgeta V, Ritchie K, Rockwood K, Sampson EL, Samus Q, Schneider LS, Selbæk G, Teri L, Mukadam N. Dementia prevention, intervention, and care: 2020 report of the Lancet Commission. Lancet. 2020;396:413–46. DOI |
8 | Fried LP, Tangen CM, Walston J, Newman AB, Hirsch C, Gottdiener J, Seeman T, Tracy R, Kop WJ, Burke G, McBurnie MA. Cardiovascular Health Study Collaborative Research Group Frailty in older adults: evidence for a phenotype. J. Gerontol. Med. Sci. 2001. DOI |
9 | Rockwood K, Andrew M, Mitnitski A. A comparison of two approaches to measuring frailty in elderly people. J Gerontol A Biol Sci Med Sci. 2007;62:738–43. DOI |
10 | Anne Gingrich A, Volkert D, Kiesswetter E, Thomanek M, Bach S, Sieber CC, and Zopf Y. Prevalence and overlap of sarcopenia, frailty, cachexia and malnutrition in older medical inpatients. BMC Geriatr. 2019;19(1):120. DOI |
11 | World Health Organization. The Top 10 Causes of Death. (accessed on 30 July 2022) Link |
12 | Katalinic A, Peters E, Beske F, Pritzkuleit R. Projection of Morbidity 2030 and 2050: Impact for the National Health System and Blood Supply. Transfus. Med. Hemother. 2010;37:155–9. DOI |
13 | Zhang T, Ren Y, Shen P, Jiang S, Yang Y, Wang Y, Li Z, and Yang Y. Prevalence and Associated Risk Factors of Cognitive Frailty: A Systematic Review and Meta-Analysis. Front. Aging Neurosci. 2021;13:755926. DOI |
14 | Solfrizzi V, Scafato E, Lozupone M, Seripa D, Giannini M, Sardone R, , Bonfiglio C, Abbrescia D, Galluzzo L, Gandin C, Baldereschi M, Di Carlo A, Inzitari D, Daniele A, Sabbà C, Logroscino G, Panza F. Additive role of a potentially reversible cognitive frailty model and inflammatory state on the risk of disability: the Italian longitudinal study on aging. Am. J. Geriatr. Psychiatry. 2017;25, 1236–48. DOI |
15 | Morley JE, Vellas B, Van Kan GA, Anker SD, Bauer JM, Bernabei R, Cesari M, Chumlea WC, Doehner W, Evans J, Fried LP, Guralnik JM, Katz PK, Malmstrom TK, McCarter RJ, Gutierrez Robledo LM, Rockwood K, von Haehling S, Vandewoude MF, Walston J. Frailty consensus: a call to action. J. Am. Med. Dir. Assoc. 2013;14:392–7. DOI |
16 | Chou His-Hsien, Lai Te-Jen Yen Chi-Hua. Sarcopenic Obesity Tendency and Nutritional Status Is Related to the Risk of Sarcopenia, Frailty, Depression and Quality of Life in Patients with Dementia. Int. J. Environ. Res. Public. Health. 2022;9(5):2492. DOI |
17 | Murman DL. The Impact of Age on Cognition. Semin. Hear. 2015;36(3):111–21. DOI |
18 | Poh L, Sim WL, Jo DG, Dinh QN, Drummond GR, Sobey CG, Li-Hsian Chen C, Lai MKP, Fann DY, and Arumugam TV. The role of inflammasomes in vascular cognitive impairment. Mol. Neurodegen. 2022;17. DOI |
19 | Almutairi MM, Sivandzade F, Albekairi TH, Alqahtani F, and Cucullo L. Neuroinflammation and Its Impact on the Pathogenesis of COVID-19. Front. Med. 2021;8:745789. DOI. |
20 | De Gaetano A, Solodka K, Zanini G, Selleri V, Mattioli AV, Nasi M, Pinti M. Molecular Mechanisms of mtDNA-Mediated Inflammation. Cells. 2021;10(11):2898. DOI |
21 | Anna Picca A, Beli R, Calvani R, Coelho-Júnior HJ, Landi F, Bernabei R, Bucci C, Guerra F, and Marzetti E. Older Adults with Physical Frailty and Sarcopenia Show Increased Levels of Circulating Small Extracellular Vesicles with a Specific Mitochondrial Signature. Cells. 2020;9(4):973. DOI |
22 | Godefroy V, Batrancourt B, Charron S, Bouzigues A, Bendetowicz D, Carle G, Rametti-Lacroux A, Bombois S, Emmanuel Cognat E, Migliaccio R, Levy R. Functional connectivity correlates of reduced goal-directed behaviors in behavioural variant frontotemporal dementia. Brain Struct. Funct. 2022;227:2971-89. DOI |
23 | Başıbüyük GÖ, Ayremlou P, Saeidlou SN, Ay F, Dalkıran A, Simzari W, Vitályos GA, Bektaş Y. A comparison of the different anthropometric indices for assessing malnutrition among older people in Turkey: a large population-based screening. J. Health Popul. Nutr. 2021;40(1). DOI |
24 | Sonti G, Ilyin ES, Plata-Salamán CR. Anorexia induced by cytokine interactions at pathophysiological concentrations. Am. J. Physiol. 1996;270(6):R1394-402. DOI |
25 | Gaudet AD, Fonken LK, Watkins LR, Nelson RJ, and Popovich PG. MicroRNAs: Roles in Regulating Neuroinflammation. The Neuroscientist. 2018;24(3):221-45. DOI |
26 | Martins TS, Trindade D, Vaz M, Campelo I, Almeida M, Trigo G, da Cruz E Silva OAB, Henriques AG. Diagnostic and therapeutic potential of exosomes in Alzheimer’s disease. J. Neurochem. 2021;156:162–81. DOI |
27 | Ke-Lu L, Hong-Yan H, Hui R, Xing-Long Y. Role of exosomes in the pathogenesis of inflammation in Parkinson’s disease. Neur. Regen. Research. 2022;17(9):1898-906. DOI |
28 | Zhang N, He F, Li T, Chen J, Jiang L, Ouyang XP, and Zuo L. Role of Exosomes in Brain Diseases. Front. Cell. Neurosci. 2021;15:743353. DOI |
29 | Xian X, Cai Li-Li, Li Y, Wang RC, Xu YH, Chen YJ, Xie YH, Zhu XL, Li YF. Neuron secrete exosomes containing miR-9-5p to promote polarization of M1 microglia in depression. J. Nanobiotechnology. 2022;20(1):122. DOI |
30 | Murton AJ, Constantin D, Greenhaff DL. The involvement of the ubiquitin proteasome system in human skeletal muscle remodelling and atrophy. Biochim. Biophys. Acta – Mol. Basis Dis. 2008;1782(12):730-43. DOI |
31 | Dalle S, Lenka Rossmeislova L, Koppo K. The Role of Inflammation in Age-Related Sarcopenia. Front. Physiol. 2017;8:1045. DOI |
32 | Rong Y-D, Bian A-L, Hu H-Y, Ma Y, and Zhou X-Z. Study on relationship between elderly sarcopenia and inflammatory cytokine IL-6, anti-inflammatory cytokine IL-10. BMC Geriatr. 2018;18: 308. DOI |
33 | Nakanishi S, Iwamoto M, Shinohara H, Iwamoto H, Kaneto H. Significance of body mass index for diagnosing sarcopenia is equivalent to slow gait speed in Japanese individuals with type 2 diabetes: Cross-sectional study using outpatient clinical data. J. Diab. Invest. 2021;12:417–24. DOI |
34 | Ting EYC, Yang AC, and Tsai SJ. Role of Interleukin-6 in Depressive Disorder. Int J Mol Sci. 2020;21(6):2194. DOI |
35 | Jo D, Yoon G, Yoen Kim O, Song J. A new paradigm in sarcopenia: Cognitive impairment caused by imbalanced myokine secretion and vascular dysfunction. Biomed. Pharmacother. 2022;147:112636. DOI |
36 | Scisciola L, Fontanella RA, Surina, Cataldo , Paolisso G, Barbieri M. Sarcopenia and Cognitive Function: Role of Myokines in Muscle Brain Cross-Talk. Life. 2021;11(2):173. DOI |
37 | Sousa-Victor P, Muñoz-Cánoves P. Regenerative decline of stem cells in sarcopenia. Mol. Aspects Med. 2016;50:109-17. DOI |
38 | Pahlavani HA. Exercise Therapy for People with Sarcopenic Obesity: Myokines and Adipokines as Effective Actors. Front. Endocrinol. 2022;13:811751. DOI |
39 | Raschke S, Eckel J. Adipo-myokines: two sides of the same coin–mediators of inflammation and mediators of exercise. Mediators Inflamm. 2013;320724. DOI |
40 | Weinstein G, Beiser AS, Choi SH, Preis SR, Chen TC, Vorgas D, Au R, Pikula A, Wolf PA, DeStefano AL, Vasan RS, Seshadri S. Serum Brain-Derived Neurotrophic Factor and the Risk for Dementia. JAMA Neurol. 2014;71(1):55–61. DOI |
41 | Munir J, Lee M, Ryu S. Exosomes in Food: Health Benefits and Clinical Relevance in Diseases. Adv. in Nutri. 2020;11:3, 687-96. DOI |
42 | Kwan P. Sarcopenia, a neurogenic syndrome? J. Aging Res. 2013:791679. DOI |
43 | Balakrishnan R, Thurmond DC. Mechanisms by Which Skeletal Muscle Myokines Ameliorate Insulin Resistance. Inter. J. Mol. Sci. 2022;23(9):4636. DOI |
44 | Laconi E, Marongiu F, and DeGregori J. Cancer as a disease of old age: changing mutational and microenvironmental landscapes. Brit. J. Canc. 2020;122:943–52. DOI |
45 | White MC, Holman DM, Boehm JE, Peipins LA, Grossman M, Henley SJ. Age and Cancer Risk: A Potentially Modifiable Relationship. Am. J. Prev. Med. 2014;46(3):S7-15. DOI |
46 | Williams GR, Dunne RF, Giri S, Shachar SS, Caan BJ. Sarcopenia in the Older Adult with Cancer. J Clin. Oncol. 2021;39(19):2068–78. DOI |
47 | Surov A, Wienke A. Prevalence of sarcopenia in patients with solid tumors: A meta-analysis based on 81,814 patients. J. Parenter. Enteral Nutr. 2022;1–8. DOI |
48 | Yee Chan M, and Siu Ho Chok K. Sarcopenia in pancreatic cancer – effects on surgical outcomes and chemotherapy. World J. Gastrointest. Oncol. 2019;15;11(7):527–37. DOI |
49 | Li C, Wu Q, Li Z, Wang Z, Tu Y, Chen C, Sun S, Sun S. Exosomal microRNAs in cancer-related sarcopenia: Tumor-derived exosomal microRNAs in muscle atrophy. Exp. Biol. Med. 2021;246(10):1156-66. DOI |
50 | Mayers JR, Wu C, Clish CB, Kraft P, Torrence ME, Fiske BP, Yuan C, Ying Bao Y, Townsend MK, Tworoger SS, Davidson SM, Papagiannakopoulos T, Yang A, Dayton TL, Ogino S, Stampfer MJ, Giovannucci EL, Qian ZR , Rubinson DA, Jing Ma J, Sesso HD, Gaziano JM, Cochrane BB, Liu S, Wactawski-Wende J, Manson JAE, Pollak MN, Kimmelman AC, Souza A, Pierce K, Wang TJ, Gerszten RE, Fuchs CS, Heiden MGV, Wolpin BM. Elevated circulating branched chain amino acids are an early event in pancreatic adenocarcinoma development. Nature Med. 2014;20:10:1193-8. DOI |
51 | Babic A, Rosenthal MH, Bamlet WR, Takahashi N, Sugimoto M, Danai LV, Morales-Oyarvide V, Khalaf N, Dunne RF, Brais LK, Welch MW, Zellers CL, Dennis C,Rifai N, Prado CM, Caan B, Sundaresan TK, Meyerhardt JA, Kulke MH, Clish CB, Ng K, Heiden MGV, Petersen GM, and Wolpin BM. Post diagnosis loss of skeletal muscle, but not adipose tissue, is associated with shorter survival of patients with advanced pancreatic cancer. Cancer Epidemiol. Biomarkers Prev. 2019;28(12):2062–9. DOI |
52 | Cole CL, Kleckner IR, Jato A, Schwarz EM, and Dunne RF. The Role of Systemic Inflammation in Cancer-Associated Muscle Wasting and Rationale for Exercise as a Therapeutic Intervention. JCSM Clin. Rep. 2018;3(2): e00065. DOI |
53 | Wang H, Hertlein E, Bakkar N, Sun H, Acharyya S, Wang J, Carathers M, Davuluri R, Guttridge DC. NF-κB Regulation of YY1 Inhibits Skeletal Myogenesis through Transcriptional Silencing of Myofibrillar Genes. Mol. Cell Biol. 2007;27(12):4374–87. DOI |
54 | Giulia Rovesti G, Valoriani F, Rimini M, Bardasi C, Ballarin R, Di Benedetto F, Menozzi R, Dominici M, and Spallanzani A. Clinical Implications of Malnutrition in the Management of Patients with Pancreatic Cancer: Introducing the Concept of the Nutritional Oncology Board. Nutrients. 2021;13(10):3522. DOI |
55 | Bodin SC, and Baehr LM. Skeletal muscle atrophy and the E3 ubiquitin ligases MuRF1 and MAFbx/atrogin-1. Am. J. Physiol. Endocrinol. Metab. 2014;15;307(6):E469–E484. DOI |
56 | Menyhért J, Wittmann G, Lechan RM, Keller E, Liposits Z, Fekete C. Cocaine- and amphetamine-regulated transcript (CART) is colocalized with the orexigenic neuropeptide Y and agouti-related protein and absent from the anorexigenic alpha-melanocyte-stimulating hormone neurons in the infundibular nucleus of the human hypothalamus. Endocrinology. 2007;148(9):4276-81. DOI |
57 | Marinho R, Alcântara PSM, OttochJP, and Seelaender M. Role of Exosomal MicroRNAs and myomiRs in the Development of Cancer Cachexia-Associated Muscle Wasting. Front. Nutr. 2017;4:69. DOI |
58 | Liu H, Qiao S, Fan X, Gu Y, Zhang Y, Huang S. Role of exosomes in pancreatic cancer. Oncol. Lett. 2021;21(4): 298. DOI |
59 | Yang J, Zhang Z, Zhang Y, Ni X, Zhang G, Cui X, Liu M, Xu C, Zhang Q, Zhu H, Yan J, Zhu VF, Luo Y, Hagan JP, Li Z, Fang J, Jatoi A, Fernandez-Zapico ME, Zheng L, Edil BH, Bronze MS, Houchen CW, Li Y-P and Li M. ZIP4 Promotes Muscle Wasting and Cachexia in Mice with Orthotopic Pancreatic Tumors by Stimulating RAB27B-Regulated Release of Extracellular Vesicles from Cancer Cells. Gastroenterology. 2019;156(3):722–34. DOI |
60 | Wang L, Zhang B, Zheng W, Kang M, Chen Q, Qin W, Li C, Zhang Y, Shao Y, Wu Y. Exosomes derived from pancreatic cancer cells induce insulin resistance in C2C12 myotube cells through the PI3K/Akt/FoxO1 pathway. Sci. Rep. 2017;7(1). DOI |
61 | Katan M, Luft A. Global Burden of Stroke. Semin. Neurol. 2018;38(2):208-11. DOI |
62 | Howard G, and Goff DC. Population shifts and the future of stroke: forecasts of the future burden of stroke. Ann. N. Y. Acad. Sci. 2012;1268:14–20. DOI |
63 | Yoo JW, Hong BY, Jo L, Kim J-S, Park JG, Shin BK, and Lim SH. Effects of Age on Long-Term Functional Recovery in Patients with Stroke. Medicina. 2020;56(9):451. DOI |
64 | Dabrowska S, Andrzejewska A, Lukomska B, and Janowski M. Neuroinflammation as a target for treatment of stroke using mesenchymal stem cells and extracellular vesicles. J. Neuroinflammation. 2019;16(1). DOI |
65 | Rose CR, Felix L, Zeug A, Dietrich D, Reiner A, Henneberger C. Astroglial Glutamate Signaling and Uptake in the Hippocampus. Front. Mol. Neuros. 2017;10:451. DOI |
66 | Yin Z, Han Z, Hu T, Zhang S, Ge X, Huang S, Wang Lu, Yu J, Li W, Wang Y, Li D, Zhao J, Wang Y, Zuo Y, Li Y, Kong X, Chen F, Lei P. Neuron-derived exosomes with high miR-21-5p expression promoted polarization of M1 microglia in culture. Brain Behav. Immun. 2020;83:270-82. DOI |
67 | Wan T, Huang Y, Gao X, Wu W, and Guo W. Microglia Polarization: A Novel Target of Exosome for Stroke Treatment. Front. Cell Dev. Biol. 2022;10:842320. DOI |
68 | Ruan L, Wang B, ZhuGe Q, and Jin K. Coupling of Neurogenesis and Angiogenesis After Ischemic Stroke. Brain Res. 2015;1623: 166–173. DOI |
69 | Zhang H, Lin S, McElroy CL, Wang B, Jin D, Uteshev VV, Jin K. Circulating Pro-Inflammatory Exosomes Worsen Stroke Outcomes in Aging. Circ. Res. 2021;129:e121–40. DOI |
70 | Morley JE. Bidirectional Communication Between Brain and Muscle. J. Nut. Health Aging. 2018;22(10):1144-5. DOI |
71 | Singh GB, Cowan DB, and Wang DZ. Tiny Regulators of Massive Tissue: MicroRNAs in Skeletal Muscle Development, Myopathies, and Cancer Cachexia. Front. Oncol. 2020;10:598964. DOI |
72 | Lam NT, Gartz M, Thomas L, Haberman M, Strande JL. Influence of microRNAs and exosomes in muscle health and diseases. J. Muscle Res. Cell Moti. 2020;41(4):269-84. DOI |
73 | Guo Y, Hong W, Wang X, Zhang P, Körner H, Tu J, and Wei W. MicroRNAs in Microglia: How do MicroRNAs Affect Activation, Inflammation, Polarization of Microglia and Mediate the Interaction Between Microglia and Glioma? Front. Mol. Neur. 2019;12:125. DOI |
74 | Pignataro G. Emerging Role of microRNAs in Stroke Protection Elicited by Remote Postconditioning. Front. Neurol. 2021;12:748709. DOI |
75 | Mahjoubin-Tehran M, Rezaei S, Jesmani A, Birang N, Morshedi K, Khanbabaei H, Khan H, Piranviseh A, Nejati M, Aschner M, Mirzaei H. New epigenetic players in stroke pathogenesis: From non-coding RNAs to exosomal non-coding RNAs. Biomed. Pharmacother. 2021;140:111753. DOI |
76 | Germanà AM, Cicciò MP, Raffa N, Randazzo G, Zagami F. Revisione critica dei biomarcatori nella diagnostica dei tumori dell’apparato digerente. G. Ital. Pat. Clin. 1991;6(2):98-104. |
77 | Vieira NF, Serafini LN, Novais PC, Neto FSL, de Assis Cirino ML, Kemp R, Ardengh JC, Saggioro FP, Gaspar AF, Sankarankutty AK, Lopes Júnior JR, da Cunha Tirapelli DP, and dos Santos JS. The role of circulating miRNAs and CA19-9 in pancreatic cancer diagnosis. Oncotarget. 2021;12(17):1638–50. DOI |
78 | Rong S, Wang L, Peng Z, Liao Y, Li D, Yang X, Nuessler AK, Liu L, Bao W, and Yang W. The mechanisms and treatments for sarcopenia: could exosomes be a perspective research strategy in the future? J. Cachexia Sarcopenia Muscle 2020;11: 348–365. DOI |
79 | Choi C. Therapeutic exosomes for various human diseases: Special issue of BMB Reports in 2022. BMB Rep. 2022;55(1): 1-2. DOI |
Cite this article:
Zagami, F. Overlapping pathophysiological pathways between sarcopenia and chronic diseases. DYSONA – Life Science, 2023;4(1): 10-22. doi: 10.30493/dls.2022.301222