Ruba D. Alsaeed 1*; Amer Q. Aldarwish 1; Lina Khouri 2; Vinothkumar Kolluru 3
1, Faculty of Engineering, Al-Wataniya Private University, Hama, Syria
2, Department of Environmental and Sanitary Engineering, Faculty of Civil Engineering, Damascus University, Damascus, Syria
3, Department of Data science, Stevens Institute of Technology, Castle Point Terrace, Hoboken, NJ 07030, USA
E-mail:
ruba.alsaeed@wpu.edu.sy
Received: 17/10/2024
Acceptance: 27/03/2025
Available Online: 30/03/2025
Published: 01/07/2025
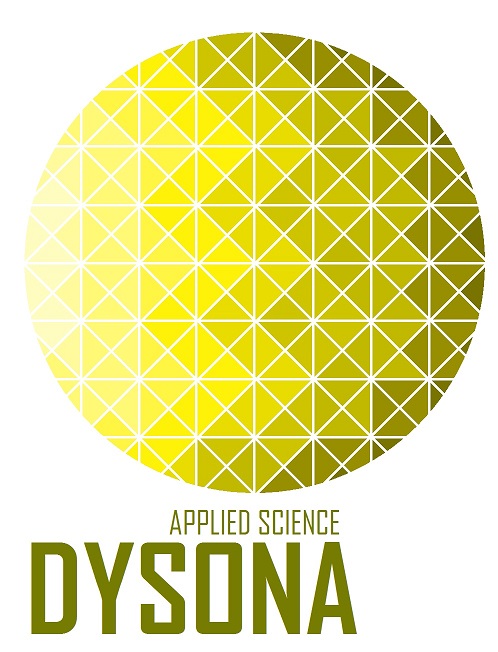
Manuscript link
http://dx.doi.org/10.30493/DAS.2025.484126
Abstract
Manganese-contaminated drinking water presents a substantial health risk to consumers. The oxidation/filtration method is regarded as an efficient technique for the removal of manganese from water. This method is affected by various factors including initial manganese concentration, oxidant concentration, pH level, aeration rate, and reaction duration. This paper presents multiple experiments to evaluate the impact of these factors on the efficiency of sodium hypochlorite (NaOCl) as an oxidant in manganese removal. The collected data was utilized to create a response surface optimization model (RSM). The findings underscore the importance of higher pH and aeration levels in improving manganese removal by oxidation, but also highlight the necessity for increased oxidant concentration to achieve strict water standards even at lower pH levels. The primary predictor was the initial Mn concentration (26.3%) followed by the concentration of the oxidant (NaOCl) (19.3%). The developed RSM exhibited significant predictive accuracy (Predicted R2=92.11%) and can be utilized in the optimization of Mn oxidation process within the investigated inputs ranges. More research is required to increase the accuracy of the developed model at different input ranges and different combinations of manganese and iron contamination levels.
Keywords: Manganese, Water, Oxidation, Sodium hypochlorite, Response surface
Introduction
Water is vital for existence and profoundly impacts the political and economic frameworks of countries. Consequently, the restricted availability of water resources has become a significant concern in formulating sustainable policies worldwide, propelled by swift population increase and economic development. In recent decades, population growth has significantly increased water use, leading to critical global challenges [1]. Surface water is frequently regarded as the primary source of potable water. Nonetheless, the persistent climate change has affected and is anticipated to continue adversely impacting the quality and quantity of surface water resources [2]. Furthermore, human activities have resulted in the contamination of the majority of surface water supplies with chemicals and contaminants [3], hence posing substantial health risks to communities. As a result, there has been an increasing dependence on groundwater supplies, particularly in developing countries and arid regions. Nonetheless, poor agricultural practices and urban runoff have exacerbated groundwater contamination with heavy and hazardous elements, raising significant concerns [4].
Manganese (Mn) is a recognized contaminant in surface and groundwater that results in water discoloration and presents substantial health risks to users. Manganese contaminates water naturally by the breakdown of iron-manganese minerals, significantly influenced by natural events such as floods and erosion [5]. On the other hand, human industrial activities, including mining [6] and the negligent and uninformed application of mineral fertilizers and pesticides [7], substantially contribute to the contamination of surface and groundwater resources with manganese. Furthermore, manganese, iron, and other elements may leach into drinking water from old, corroded iron pipes [8]. Consequently, resolving the issue of manganese contamination necessitates a comprehensive study of its various sources, alleviating its harmful health effects, and formulating decontamination solutions for drinking water.
Manganese decontamination in drinking water can be effectively accomplished using many processes, including oxidation/filtration, chemical precipitation, coagulation, flotation, ion-exchange, electrochemical treatment, adsorption, and membrane filtration [9]. The oxidation/filtration process is likely one of the most prevalent and effective techniques for removing manganese from water. This method employs a strong oxidant, such as sodium hypochlorite (NaOCl) or potassium permanganate (KMnO4), to oxidize soluble manganese into insoluble manganese dioxide (MnO2), subsequently followed by filtration to eliminate the precipitated MnO2 particles [10]. In this context, NaOCl is deemed more effective than KMnO4 [11], particularly when the oxidation process occurs under enough aeration followed by an efficient filtration procedure [12].
Multiple parameters may affect the manganese oxidation process and sodium hypochlorite efficacy in manganese removal from water, including initial manganese concentration, aeration levels, pH, duration of the oxidation process, and oxidant concentration. This research investigates the factors affecting manganese removal efficiency via the sodium hypochlorite oxidation process and aims to offer a response surface optimization model to facilitate real-time process enhancement in water purification facilities.
Material and Methods
The experiments
The experiment was conducted at the Homs Water Department Laboratory (Homs, Syria). A total of four manganese oxidation water experiments were performed as detailed in Table 1. All tests relied on the principle of soluble manganese oxidation utilizing sodium hypochlorite (NaOCl) to produce insoluble manganese dioxide, which may be eliminated via standard filtration systems in accordance with the formula:
Mn2+ + NaOCl + 2H2O → MnO2↓ + Na+ + 2H+ + HOCl

1st experiment (pH effect on NaOCl efficiency in Mn oxidation):
In this experiment, an initial concentration of Mn (1.5 mg/L) was achieved by dissolving 3.076 g Manganese sulfate (MnSO4·H2O) in 1 L of distilled water (1000 mg/L Mn). Then, 150 ml of this stock was completed with distilled water to 100 L to reach a final manganese concentration of 1.5 mg/L. Various levels of pH (6, 7, 8, 9, and 10) were achieved using HCl (1N) and NaOH (1N) utilizing a calibrated pH meter.
After preparing the needed experimental water adjusted to the required pH level, oxidant (NaOCl) was added at various levels (1, 1.5, 2, and 2.5 mg/L) by pipetting 20, 30, 40, and 50 μL of sodium hypochlorite (5%) to a volumetric flask containing approximately 1 L of pH adjusted water samples. The volume was then completed to 1 L using similar water and the flask contents were transferred to a Jar test apparatus. mixing was carried out at a speed of 200 rpm for 3 minutes, followed by slow mixing at 30 rpm, to ensure sufficient contact time for NaOCL with the samples. After a contact time of 5 minutes, the samples were left to settle and MnO2 to form, then the residual Mn analyses were carried out at 30 minutes intervals (30, 60, and 90 minutes) after filtration using filter paper to simulate conventional filtration systems.
2nd experiment (Dissolved oxygen effect on NaOCl efficiency in Mn oxidation):
Similar approach was used in this experiment to prepare water samples with an initial Mn concentration of 1.5 mg/L and pH of 7.5. Similar oxidant levels of 1, 1.5, 2, and 2.5 mg/L were used. As for dissolved oxygen (DO) settings, 3W/3.5 L/min aeration pump (AB-510) was used to achieve various levels of aeration (DO= 4, 5, 6, and 7 mg/L) in each jar test apparatus. DO level was kept regulated and monitored using a portable dissolved oxygen meter (HQ1130 Hach®). The residual Mn was analyzed at 10 minutes intervals until after 1 hour of reaction.
3rd experiment (Initial Mn concentration effect on NaOCl efficiency in Mn oxidation):
In this experiment, water samples with various initial Mn concentrations (1.5, 2, 2.5, 3, and 3.5 mg/L) were prepared utilizing a manganese stock similar to that in the first experiment with 30, 40, 50, and 60 ml of the stock used to prepare 20 L of each concentration, respectively. NaOCl was added at various levels (1, 1.5, 2, 2.5, and 3 mg/L) by pipetting 20, 30, 40, 50, and 60 μL of sodium hypochlorite (5%) to a volumetric flask containing 1 L of pH adjusted water samples. The samples were then transferred to the jar test apparatus and treated similar to the first experiment, with the residual Mn being analyzed after 60 minutes.
4th experiment (Iron concentration effect on NaOCl efficiency in Mn oxidation):
In this experiment, an initial Mn concentration of 1.5 m/L was prepared. Then, a ferrous chloride (FeCl₂) 1000 mg/L stock was prepared by dissolving 0.436 g of FeCl2in 100 ml of distilled water. Different Fe concentrations (0, 1, 1.5, and 2 mg/L) water samples were prepared utilizing FeCl2 with 0, 20, 30, and 40 ml of the stock used to prepare 20 L of each concentration, respectively. NaOCl concentration, experiment setting, and the residual Mn analysis was conducted similar to the 3rd experiment.
Residual Mn analysis
The general potassium iodide (KI) reagent colorimetric procedure was utilized to measure the residual Mn concentration in all experiments [13]. For that purpose, 0.5 ml of concentrated H2SO4 was added to each 2.5 ml tested water sample or standard solution. Then, 2.5 ml of KI reagent was added to the acidified sample. The mixture was left for 5 minutes until full color development, and the absorption value at 425 nm was measured in a single-beam spectrophotometer (DR/2010 Hach®). Mn concentration was then calculated using the absorption readings compared to a standard curve.
Response surface regression model (RSM)
Response surface regression model was developed utilizing the data collected from all experiments except for iron concentration effect experiment (4th experiment) since there were no enough data to include iron as a predictor. Therefore, the participating predictors were initial Mn concentration (IMn), pH, dissolved oxygen (DO), oxidation time (T), and oxidant concentration (OC). The residual Mn concentration (FMn) was assigned as the targeted response. Minitab® 19 software was used to conduct RSM analysis.
Results and Discussion
The effect of pH on the efficiency of sodium hypochlorite in manganese oxidation
This experiment evaluated several pH levels to determine the efficacy of various NaOCl concentrations in oxidizing and removing soluble manganese from water (Supplementary Table 1). The results were juxtaposed with the recommended manganese limits in drinking water as per LHA (Mn<0.3 mg/L) [14] and SMCL (Mn<0.05 mg/L) [15], and contour plotting was employed to illustrate the final outcomes (Fig. 1).
The initial 20 minutes of the reaction were the most crucial, leading to the most significant reduction in residual Mn concentration, irrespective of the NaOCl concentration and pH level (Fig. 1). The findings demonstrated that the final removal efficiency for 1 mg/L of NaOCl was 55.33%, 73.33%, 80%, 86.67%, and 91.33% at pH levels of 6, 7, 8, 9, and 10, respectively, with an initial manganese concentration of 1.5 mg/L. The percentages were significantly increased to 86.67%, 93.33%, 97.33%, 100%, and 100%, respectively, for the NaOCl dosage of 2.5 mg/L (Supplementary Table 1).
The LHA standard was achieved at lower NaOCl concentrations (1 and 1.5 mg/L) solely at pH levels above 8 and 7, respectively (Fig. 1 A and B), whereas comparable limits were seen at a pH of 6 with 2 mg/L of NaOCl (Fig. 1 C). LHA standards were achieved in less than 60 minutes, irrespective of pH level, when NaOCl was applied at a concentration of 2.5 mg/L. Furthermore, SMCL standards were met in this treatment, albeit at pH levels exceeding 9 (Fig. 1 D) (Supplementary Table 1).
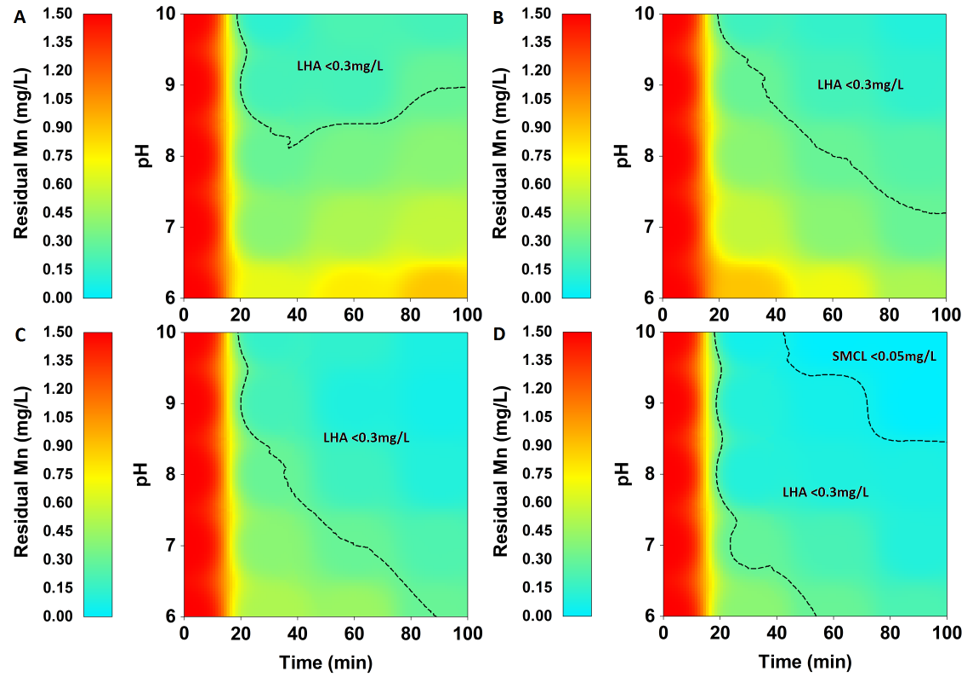
No definitive guidelines exist about the permissible concentration of Mn in potable water [16]. Higher manganese exposure in drinking water is linked to neurological problems, rendering infants and children more susceptible to manganese concentrations than adults [17]. The Environmental Protection Agency of the United States (EPA U.S) has established a lifetime health advisory (LHA) that sets the manganese (Mn) level in drinking water at 0.3 mg/L, deemed safe for consumption without adverse health effects [14]. The Secondary Maximum Contaminant Level (SMCL) establishes a non-enforceable manganese level of 0.05 mg/L, which does not negatively impact the color or flavor of drinking water [15]. The present findings underscore the significance of pH levels in facilitating manganese removal by oxidation; nevertheless, they also emphasize the necessity for elevated concentrations of oxidant (NaOCl) to expedite the oxidation process and comply with rigorous water quality regulations, even at reduced pH levels.
The effect of aeration on the efficiency of sodium hypochlorite in manganese oxidation
Various aeration levels (DO = 4, 5, 6, and 7 mg/L) in combination with various oxidant levels were investigated to assess the efficiency of Mn removal under constant pH of 7.5 and constant initial Mn concentration of 1.5 mg/L. the experiment was monitored at 10 minutes interval up to 1 hour of oxidation, and the final removal efficiency was calculated accordingly.
It was noted that the final manganese removal efficiency increases with increasing DO value. Raising DO value from 4 to 7 mg/L raised the final Mn removal efficiency from 57.33% to 83.33% under NaOCl application of 1 mg/L. However, at higher NaOCl concentration (2.5 mg/L), the final Mn removal efficiency was increased only from 90.67% to 100% (Supplementary Table 2).
Contour plotting indicates that under the lowest NaOCl concentration (1 mg/L), Mn removal was notably gradual, and LHA standards can only be met at higher aeration levels (DO>6 mg/L) and after approximately 1 hour of reaction (Fig. 2 A). On the other hand, the same limits can be met more rapidly by doubling NaOCl concentrations (2 mg/L) and regardless of aeration level (DO>4 mg/L) (Fig. 2 C). The strict SMCL standard was only achieved under the highest NaOCl concentration (2.5 mg/L) combined with high aeration levels (DO>5.5 mg/L) (Fig. 2 D) (Supplementary Table 2).
These observations indicate that aeration is crucial in enhancing oxidation efficiency, especially under low NaOCl concentrations. However, it is more important to increase oxidant concentration in order to meet higher water standards.
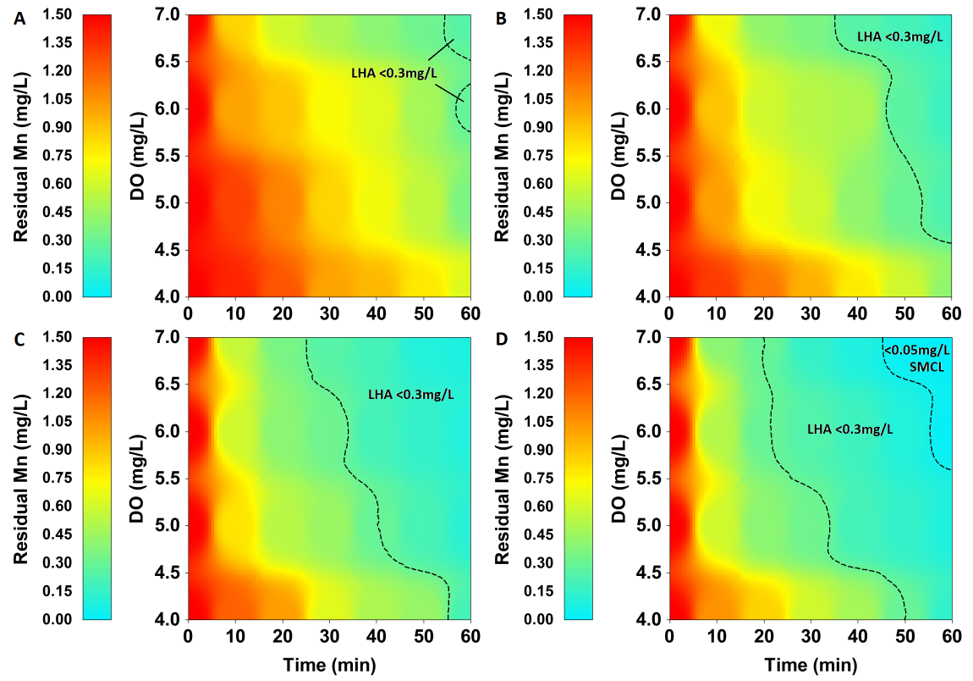
The effect of initial Mn concentration on Mn oxidation
In this setting, constant aeration and pH levels were constant (DO=5 mg/L, pH=7.5) and the effect of various Mn input (initial concentration) and oxidant (NaOCl) levels were tested in combination. The results show that a concentration of NaOCl similar to that of initial Mn is required to achieve LHA limits (Mn<0.3 mg/L) (Supplementary Table 3). This observation was also visualized through contour plotting (Fig. 3) with SMCL standard reached only using a NaOCl concentration of 3 mg/L with an initial Mn concentration of 1.5 mg/L.
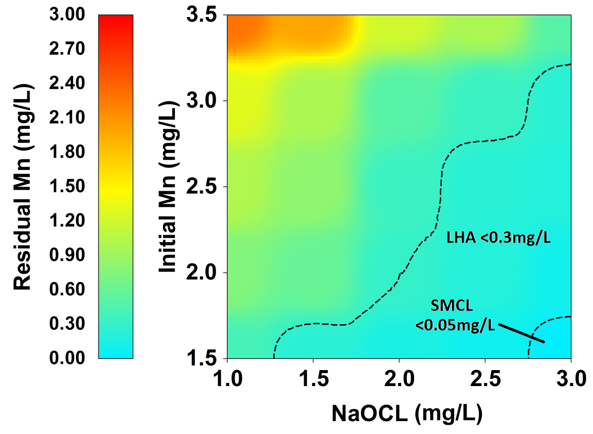
The influence of companion iron concentration manganese oxidation
In this experiment, various concentrations of Fe and oxidant (NaOCl) were tested to assess the efficiency of Mn removal while maintaining constant aeration (DO=5 mg/L), pH (7.5), time (60 minutes), and initial Mn concentration (1.5 mg/L). The results showed that Fe cation compromised Mn oxidation process, especially under high concentration. In fact, Mn removal efficiency decreased from 80% in the absence of Fe to only 20% when Fe concentration reached 3 mg/L taking into consideration a constant oxidant concentration of 1 mg/L (Fig. 4) (Supplementary Table 4).
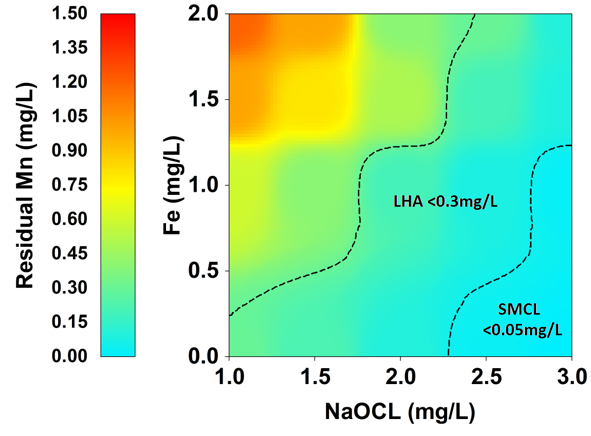
Iron and manganese frequently coexist in water due to analogous contamination sources, and both can be removed through oxidation, transforming their soluble forms (Fe2+ and Mn2+) into insoluble forms (Fe3+ and Mn4+) that can be filtered out [17]. Consequently, the present observation of heightened NaOCl dosage necessity for Mn elimination is warranted, as both elements compete for the oxidizing agent [19]. Consequently, it is essential to monitor the initial concentration of Fe and Mn to accurately ascertain the required oxidant concentration, necessitating further research to evaluate various combinations of contaminant inputs and utilized oxidants.
Response surface regression model (RSM) results
Petro chart of the standardized effect showed that initial Mn concentration was the most influential single predictor (26.3%) followed by oxidant (NaOCl) concentration (19.3%), time (14.2%), pH (10.9%), and dissolved oxygen (8.9%). It is worth noting that the interaction effect of time (T*T) and initial Mn with oxidant concentrations (IMN*OC) recorded high effects of 12.2% and 11.8%, respectively (Fig. 5). The adjusted R2 for the developed RSM was 93.32% and with a predicted R2 of 92.11% highlighting the accuracy and generalization of the model, since both values are almost similar [20]. The final regression equation for the developed model was:
FMN = 7.275 – 0.197×IMn – 0.468×pH – 0.815×DO – 0.04722×T – 0.679×OC + 0.2741×IMn*IMn + 0.01141×pH*pH + 0.05088×DO*DO + 0.000163×T*T + 0.0702×OC*OC – 0.3064×IMn*OC + 0.001178×pH*T + 0.0584×pH*OC + 0.002123×DO*T + 0.0213×DO*OC + 0.001890×T*OC
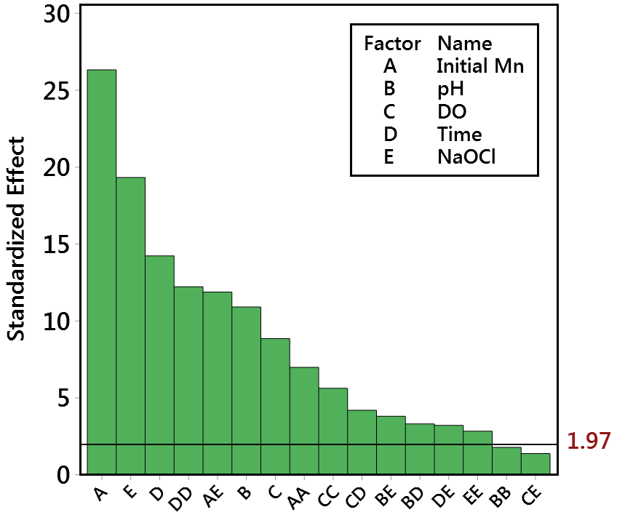
Where (FMn) is the final Mn concentration, (IMn) is the initial Mn concentration, (DO) is the dissolved oxygen, (T) is oxidation time, and (OC) is oxidant concentration. The resulting equation was utilized in optimizing Mn oxidation conditions to achieve LHA and SMCL limits within the investigated experimental ranges (Table 2).
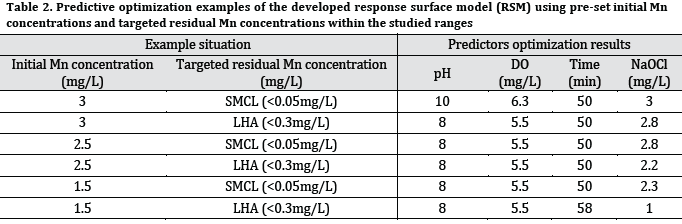
Study limitation and future research recommendations
The developed response surface regression model can be highly beneficial in developing real life strategies in water treatment, as it is a well-known tool in water treatment modeling [21]. However, predicting in the most extreme values of used oxidant, aeration, and pH levels might be inaccurate. In fact, RSM can produce low accuracy predictions under these circumstances, as the flexibility and realism of the functions may degrade near extreme regions of the factor space [22]. Therefore, the provided sample predictions (Table 2) were selected to be within the tested ranges of data inputs. Consequently, other combinations and different experimental settings and ranges are recommended for future studies in order to increase the accuracy of developed models.
Minor differences can be observed between experiments (such as 3rd and 4th experiments) under similar conditions. For instance, Mn removal efficiency in the 3rd experiment was slightly higher (although comparable) than that of the 4th experiment under the same initial Mn (1.5 mg/L) and oxidant concentrations (Supplementary Tables 3 and 4). Such differences might be attributed to other non-controlled or non-investigated factors (such as temperature) which might have influenced the final removal efficiency results. Therefore, it is suggested that these factors are taken into consideration in future research in order to incorporate it into the final RSM model (or any other model) and increase its accuracy and applicability in practice.
Conclusions
The study evaluated the efficacy of varying concentrations of NaOCl in the oxidation and removal of soluble manganese from water. The findings underscore the significance of increased pH and aeration levels in improving manganese removal by oxidation, while simultaneously emphasizing the necessity for elevated oxidant concentrations to comply with stringent water quality regulations, even at reduced pH levels. The results of the response surface regression model (RSM) indicated that starting manganese concentration was the most significant predictor, succeeded by oxidant concentration, time, pH, and dissolved oxygen. The model may assist in formulating practical water treatment solutions; however, more research is required to increase the accuracy of the developed model under wider ranges of predictors.
References
- Alsaeed RD, Alaji B, Ibrahim M. Predicting aluminium using full-scale data of a conventional water treatment plant on Orontes River by ANN, GEP, and DT. Int. J. Water. 2023;15(3):190-206. DOI
- Whitehead PG, Wilby RL, Battarbee RW, Kernan M, Wade AJ. A review of the potential impacts of climate change on surface water quality. Hydrol. Sci. J. 2009;54(1):101-23. DOI
- Kjellstrom T, Lodh M, McMichael T, Ranmuthugala G, Shrestha R, Kingsland S. Air and water pollution: burden and strategies for control. Disease Control Priorities in Developing Countries. 2nd edition. 2006.
- Mustafa BM, Hassan NE. Water contamination and its effects on human health: A review. J. Geogr. Environ. Earth Sci. Int. 2024;28(1):38-49. DOI
- Li J, Zhang C, Tan H, Zeng M, Cheng Y. Occurrence and influencing factors of high groundwater manganese in the oxbow lakes of the middle reaches of Yangtze River. J. Hydrol. 2024;630:130713.
- Matveeva VA, Alekseenko AV, Karthe D, Puzanov AV. Manganese pollution in mining-influenced rivers and lakes: Current state and forecast under climate change in the Russian Arctic. Water. 2022;14(7):1091. DOI
- Rad SM, Ray AK, Barghi S. Water pollution and agriculture pesticide. Clean Technol. 2022;4(4):1088-102. DOI
- Alvarez-Bastida C, Martínez-Miranda V, Solache-Ríos M, Linares-Hernández I, Teutli-Sequeira A, Vázquez-Mejía G. Drinking water characterization and removal of manganese. Removal of manganese from water. J. Environ. Chem. Eng. 2018;6(2):2119-25. DOI
- Patil DS, Chavan SM, Oubagaranadin JUK. A review of technologies for manganese removal from wastewaters. J. Environ. Chem. Eng. 2016;4(1):468–87. DOI
- Kouzbour S, El Azher N, Gourich B, Gros F, Vial C, Stiriba Y. Removal of manganese (II) from drinking water by aeration process using an airlift reactor. J. Water Process Eng. 2017;16:233-9. DOI
- Zhang Y, Dong H, Yan P, Zheng X. Research on removal of manganese in drinking water by potassium permanganate. InE3S Web of Conferences. EDP Sciences. 2021;260:01025. DOI
- Kan CC, Chen WH, Wan MW, Phatai P, Wittayakun J, Li KF. The preliminary study of iron and manganese removal from groundwater by NaOCl oxidation and MF filtration. Sustain. Environ. Res. 2012;22(1):25-30.
- Tighe M, Edwards MM, Cluley G, Lisle L, Wilson SC. Colorimetrically determining total antimony in contaminated waters and screening for antimony speciation. J. Hydrol. 2018;563:84-91. DOI
- USEPA. Health effects support document for manganese (EPA 822-R-03-003). 2003. Available from: Link (Accessed 20 Mar. 2025).
- USEPA. Drinking Water Health Advisory for Manganese. 2004. Available from: Link (Accessed 20 Mar. 2025).
- Friedman A, Boselli E, Ogneva-Himmelberger Y, Heiger-Bernays W, Brochu P, Burgess M, Schildroth S, Denehy A, Downs T, Papautsky I, Clauss Henn B. Manganese in residential drinking water from a community-initiated case study in Massachusetts. J. Expo. Sci. Environ. Epidemiol. 2024;34(1):58-67. DOI
- Balachandran RC, Mukhopadhyay S, McBride D, Veevers J, Harrison FE, Aschner M, Haynes EN, Bowman AB. Brain manganese and the balance between essential roles and neurotoxicity. J. Biol. Chem. 2020;295(19):6312-29.
- Ayoub M. Removal of iron and manganese by the fabric capillary action. Int. J. Eng. Sci. 2018;7(10):28-31.
- Ellis D, Bouchard C, Lantagne G. Removal of iron and manganese from groundwater by oxidation and microfiltration. Desalination. 2000;130(3):255-64. DOI
- Sai DA, Nataraj KS, Lakshmana RA. Response surface methodology-a statistical tool for the optimization of responses. Glob. J. Addict. Rehabil. Med. 2023;7(1):555705. DOI
- Nair AT, Makwana AR, Ahammed MM. The use of response surface methodology for modelling and analysis of water and wastewater treatment processes: a review. Water Sci. Technol. 2014;69(3):464-78. DOI
- Downing DJ, Gardner RH, Hoffman FO. An examination of response-surface methodologies for uncertainty analysis in assessment models. Technometrics. 1985;27(2):151-63. DOI
Cite this article:
Alsaeed, R. D., Aldarwish, A. Q., Khouri, L., Kolluru, V. Response surface modeling of sodium hypochlorite-based manganese oxidation in drinking water. DYSONA – Applied Science, 2025;6(2): 334-342. doi: 10.30493/das.2025.484126